Thank you for visiting nature.com. You are using a browser version with limited support for CSS. To obtain the best experience, we recommend you use a more up to date browser (or turn off compatibility mode in Internet Explorer). In the meantime, to ensure continued support, we are displaying the site without styles and JavaScript.
Scientific Reports volume 12, Article number: 13880 (2022 ) Cite this article Urolithin A
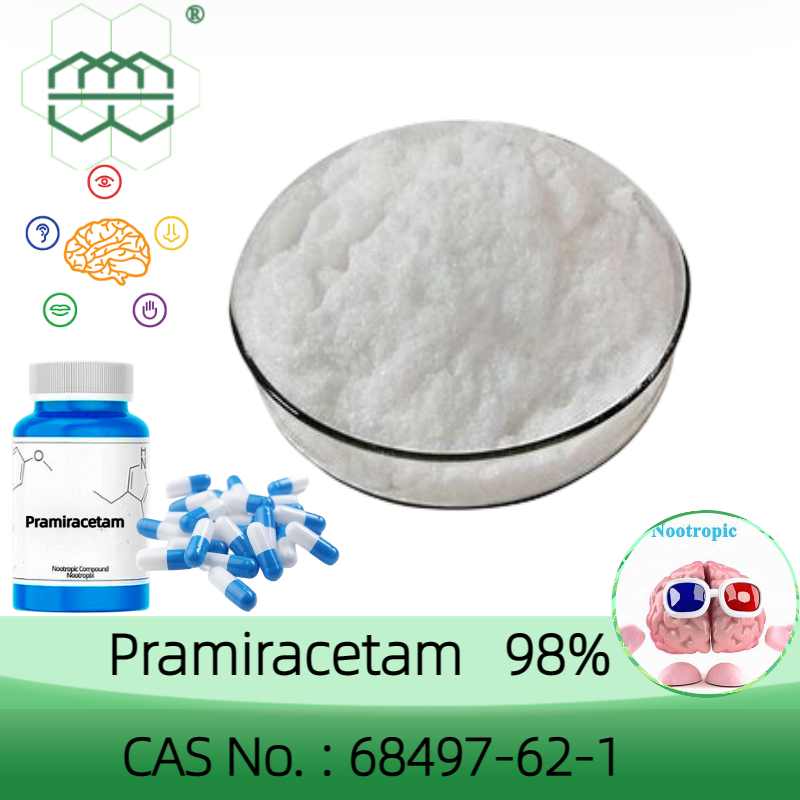
A series of 1″-(alkylsulfonyl)-dispiro[indoline-3,2′-pyrrolidine-3′,3″-piperidine]-2,4″-diones 6a‒o has been synthesized through regioselective multi-component azomethine dipolar cycloaddition reaction of 1-(alkylsulfonyl)-3,5-bis(ylidene)-piperidin-4-ones 3a‒h. X-ray diffraction studies (6b‒d,h) confirmed the structures. The majority of the synthesized analogs reveal promising antiproliferation properties against a variety of human cancer cell lines (MCF7, HCT116, A431 and PaCa2) with good selectivity index towards normal cell (RPE1). Some of the synthesized agents exhibit potent inhibitory properties against the tested cell lines with higher efficacies than the standard references (sunitinib and 5-fluorouracil). Compound 6m is the most potent. Multi-targeted inhibitory properties against EGFR and VEGFR-2 have been observed for the synthesized agents. Flow cytometry supports the antiproliferation properties and shows the tested agents as apoptosis and necrosis forming. Vero cell viral infection model demonstrates the anti-SARS-CoV-2 properties of the synthesized agents. Compound 6f is the most promising (about 3.3 and 4.8 times the potency of the standard references, chloroquine and hydroxychloroquine). QSAR models explain and support the observed biological properties.
Compounds containing the spiro-indole framework occupy a unique place in the heterocyclic space that spans pharmaceutical and natural alkaloids1,2. Synthesis of spiro-heterocycles through the reactive carbonyl group is a subject of major interest for organic researchers3. Diverse synthetic methodologies have been reported for spiro-heterocycles, including intermolecular alkylation4,5, Morita–Baylis–Hillman6,7, 1,3-dipolar cycloaddition8,9, Mannich/Pictet–Spengler3,10, sigmatropic rearrangement11,12 and electrocyclization13 reactions. Many natural spiro-indoles with considerable biological properties have been identified, of which maremycin G (isolated from Streptomyces sp. B9173)14,15, maremycin F (isolated from Streptomyces sp. GT051237)15,16 spirotryprostatins A and B (isolated from Aspergillus fumigatus’ fermentation broth)17, strychnofoline (isolated from Strychnos usambarensis)18,19 and surugatoxin (isolated from ivory shell)20 reveal antimitotic activity (Fig. 1). Additionally, many synthetic spiro-indole analogs display considerable antimicrobial21,22,23,24, antitumor8 and cholinesterase inhibitory properties25,26,27,28.
The current study is directed towards synthesis and investigation of the biological properties of novel 3-spiro-indolin-2-ones prepared through azomethine dipolar cycloaddition to the exocyclic olefinic linkage of 3,5-bis(arylidene)-N-sulfonyl-4-piperidones. Interest in conjugation of the sulfonyl group to the heterocyclic nitrogen of 4-piperidone forming a sulfonamide fragment is due to the distinct physicochemical properties of the oxygen-rich sulfonyl residue that may increase the hydrophilicity of the bio-active agent29,30. Sulfonamides were flagged as attractive bio-active targets long ago (Gerhard Domagk, 1935) due to their antibacterial properties31. The sulfonamide motif can tether the bioactive agent in the targeted receptor/protein by forming hydrogen bonding with the neighboring amino acid functions. It is usually recognized as the bioisostere of the carboxylic group with limited drawbacks relative to the latter (metabolic toxicity, instability and capability for diffusion through bio-membranes)31,32. Many sulfonamides exert high efficacy as anticancer agents and have been approved as therapeutics. Belinostat [approved by Food and Drug Administration (FDA) in 2014] is used for treatment of peripheral T-cell lymphoma as a histone deacetylase inhibitor33,34. Vemurafenib (Zelboraf, approved by FDA in 2011 and 2017) treats BRAF V600 mutated late-stage skin cancer and Erdheim-Chester disease35,36. Dabrafenib (Tafinlar, approved in 2013 and 2018) is used for treatment of advanced melanoma and Mekinist also treats BRAF-positive cancer37,38 (Fig. 2). Many sulfonamide candidates have been reported as potential antitumor agents due to their aromatase, topoisomerase or carbonic anhydrase inhibitory properties31,39,40,41.
Cancer is one of the most severe diseases threatening human life. Although many methodologies and techniques in addition to numerous drugs have been discovered and approved for cancer treatment, millions of people continue to suffer from the illness every year. The off-target effect is one of the major drawbacks of cancer therapeutics42. Consequently, recent focus in cancer chemotherapy is on the development of highly selective anticancer agents devoid of off-target effects, thereby producing enhanced potency/efficacy towards the cancer cell with reduced side effects31.
Owing to the anti-SARS-CoV-2 (severe acute respiratory syndrome coronavirus-2) properties of indole43,44,45,46,47,48 and sulfonamide-containing compounds49 the targeted agents within the current study are also considered for anti-SARS-CoV-2 investigation. In the beginning of 2020, a health-socio-economic disaster emerged globally due to the highly infectious disease. Severe acute respiratory syndrome due to pathogenic viral infection of SARS-CoV-2 leads to COVID-19 (coronavirus disease 2019). It is postulated that viral zoonotic jump (probably bat) to human was firstly recognized in Wuhan, China. The infection then spread worldwide causing a universal pandemic, according to WHO (World Health Organization) in March 202050,51. Lack of an effective therapeutic was a major factor in the ensuing threat to human life. Several symptoms are associated with infection of which a cough, running noise, loss of smell and sometimes fever were highly publicized. In severe cases, blood clotting disorders and stroke are observed52. Drug repurposing was a wise and rapid strategy for urgent identification of potential therapeutics capable of controlling the disaster and saving lives. Usually, identification of novel drugs (de novo approach) with successive testing at pre-clinical and clinical phases takes several years. On the other hand, drug re-purposing allows faster adoption and utilization of well-studied existing and accessible therapeutics for treatment of infected patients53. Recently, Paxlovid (combination of Nirmatrelvir and Ritonavir) and Molnupiravir (Lagevrio) were approved (Dec. 2021) by the FDA emergency use authorization54,55,56,57 (Fig. 3). Recent publications have mentioned the effective treatment of COVID-19 patients with anticancer drugs58,59. The successful clinical trials of colon cancer patients with antiviral drugs alone or in combination with anticancer drugs60, also inspired the biological studies considered in the current work. Reported are the results of investigation of antiproliferation (against cancer cell lines) and antiviral (anti-SARS-CoV-2) properties of the targeted spiro-heterocycles with particular focus on safety-related effects on normal cells. Arbidol which is an indolyl scaffold (Fig. 3) and been used as anti-influenza drug, was recently re-purposed against SARS-CoV-261,62,63,64,65. This is encouraging regarding the prospect of using the compounds from the current investigation against SARS-CoV-2 in addition to anti-tumor properties based on the mentioned bio-properties of the chemical scaffold considered.
Drugs for treatment of COVID-19.
The 1-(alkylsulfonyl)-3,5-bis(ylidene)-piperidin-4-ones 3a‒h were obtained through dehydrohalogenation of the alkane sulfonyl chloride 2a,b with the corresponding 3,5-bis(ylidene)-4-piperidinones 1a‒e in dry tetrahydrofuran (THF) in the presence of triethylamine (TEA)66.
The azomethine ylide was obtained in situ through condensation of sarcosine 5 (secondary amino acid) and the appropriate isatin 4a,b.
Multi-component dipolar cycloaddition reaction of 3a‒h and azomethine ylide in refluxing ethanol afforded the targeted (E)-1″-(alkanesulfonyl)-4′-aryl-5″-arylidene-1′-methyl-dispiro[indoline-3,2′-pyrrolidine-3′,3″-piperidine]-2,4″-dione 6a‒o (Fig. 4). Chemical structures of the synthesized agents were characterized by various spectroscopic techniques (IR, 1H-NMR and 13C-NMR) and elemental analysis data in addition to X-ray single crystallographic studies of representative examples (6b‒6d and 6h). The IR spectrum of compound 6a reveals the indolyl NH at ν = 3186 cm−1. The piperidinyl and indolyl carbonyls are observed at ν = 1705 and 1678 cm−1, respectively. The upfield protons of the diastereotopic piperidinyl H2C-2″ and H2C-6″ appear as doublet signals at δH = 2.25 and 3.54, respectively. The downfield protons of the piperidinyl H2C-2″ and H2C-6″ are overlapped as a multiplet signal at δH = 3.93‒3.98. The pyrrolidinyl H2C-5′ protons are also diastereotopic at δH = 3.37, 3.84 while the pyrrolidinyl methine proton HC-4′ is seen as a triplet signal at δH = 4.71. The 13C-NMR spectrum of 6a shows the piperidinyl methylene carbons H2C-6″ and H2C-2″ at δC = 46.5 and 47.9, respectively. The pyrrolidinyl HC-4′, H2C-5′ carbons are observed at δC = 45.7 and 57.3, respectively. The spiro carbons C-3′ (C-3″), C-3 (C-2′) are located at δC = 61.2, 75.3, respectively. 1H, 1H-Cosy and HSQC spectra of a representative example (6f) support the assignments mentioned (Supplementary Figs. S1‒S47).
Synthesis of the targeted compounds 6a‒o.
Molecular structures of 6b, 6c, 6d and 6h are shown in Fig. 5 with structure determination presented in Supplementary Table S1. A possible factor in the ability of the molecules to interact with biological systems is molecular flexibility, in which the rotational freedom of the alkane-sulfonyl and phenyl groups would play a role. The geometry of the pyrrolidine-piperidine systems in all the crystals of 6b, 6c and 6d is very similar, as indicated by the torsion angles (Supplementary Table S2). The molecule in the crystal structure of 6h also has generally similar geometry but significant deviation, of over 20° from the nearest value for the other molecules, for angle 4–5–6–1. This indicates that some flexibility is possible for the pyrrolidine-piperidine ring system. It is notable that the crystal of 6h contains a solvate molecule (ethanol).
The molecular structures of (a) 6b, (b) 6c (c) 6d and (d) 6h.
Antiproliferation properties of the targeted agents were investigated by the standard MTT technique against diverse human cancer cell lines [MCF7 (breast), HCT116 (colon), A431 (skin squamous) and PaCa2 (pancreatic)]67 (Table 1, Supplementary Figs. S48‒S51). 5-Fluorouracil (a clinically accessible drug for colon, breast and skin cancers)68,69 and sunitinib (an effective drug for gastrointestinal, renal and pancreatic cancers)70,71 were considered as standard references.
Many of the synthesized spiro-3-indolin-2-ones reveal promising antiproliferation potency against MCF7 cancer cell line. Compound 6m (R = 4-BrC6H4, R′ = Me, R″ = H) is the most effective agent with antiproliferation properties close to 5-fluorouracil and higher than sunitinib (IC50 = 3.597, 3.15, 3.97 µM for 6m, 5-fluorouracil and sunitinib, respectively). Compound 6l (R = 4-ClC6H4, R′ = Et, R″ = Cl) also shows comparable antiproliferation efficacy (IC50 = 3.986 µM). Compounds 6f, h‒k display considerable potency as anti-MCF7 as well (IC50 = 4.375‒5.014 µM).
Some SARs (structure–activity relationships) can be assigned due to the observed antiproliferation properties. The chloro-substituted indolyl-containing heterocycles are more effective anti-MCF7 agents than the unsubstituted analogs (compound 6o is an exception). The efficacy of halo-substituted phenyl containing compounds as anti-MCF7 is in the following order bromophenyl > chlorophenyl > fluorophenyl suggesting the inductive effect (‒I effect) of the halogen atom as a collaborative factor for antiproliferation properties. The higher the ‒I effect of the halogen atom, the lower antiproliferation properties against MCF7 as shown in compounds 6m/6i/6e (IC50 = 3.597, 5.014, 15.532 µM, respectively), 6j/6f (IC50 = 4.514, 5.000 µM, respectively), 6k/6g (IC50 = 4.375, 10.319 µM, respectively) and 6l/6h (IC50 = 3.986, 4.694 µM, respectively).
Generally, all the synthesized agents (compound 6o is an exception) reveal enhanced anti-HCT116 properties exceeding that of 5-fluorouracil (a potent drug against colon cancer). Compound 6m (R = 4-BrC6H4, R′ = Me, R″ = H) heads the synthesized analogs with higher efficacy against HCT116 than those of the standard references used (IC50 = 3.236, 20.43, 9.67 µM for 6m, 5-fluorouracil and sunitinib, respectively). Compounds 6g,h,j‒l also show promising efficacies against HCT116 (IC50 = 4.111‒4.944 µM).
SARs inferred from the anti-HCT116 results are similar to those mentioned for the anti-MCF7 observations. The chloroindolinyl-containing heterocycles (compound 6o is an exception) have higher anti-HCT116 properties than the unsubstituted derivatives. Additionally, the –I effect due to the halogen atom attached to the phenyl ring is an important parameter for anti-HCT116 properties. It is notable that the fluorophenyl-containing compounds have lower anti-HCT116 properties than chlorophenyl-containing analogues. However, the bromophenyl-containing analogue (6m) is the most effective/leading agent relative to the other halogenated phenyl-containing compounds. This SAR trend is supported by the antiproliferation observations for compounds 6m/6i/6e (IC50 = 3.236, 5.472, 9.894 µM, respectively), 6j/6f (IC50 = 4.722, 5.431 µM, respectively), 6k/6g (IC50 = 4.167, 4.944 µM, respectively) and 6l/6h (IC50 = 4.111, 4.597 µM, respectively).
Compound 6m is the most promising agent synthesized with efficacy about 9.6 times more than the standard reference (IC50 = 2.434, 23.44 µM for 6m and 5-fluorouracil, respectively). Comparable potency was also displayed by compound 6k (IC50 = 2.966 µM). Compounds 6d,f,i,j,l additionally show promising efficacies (IC50 = 3.694‒4.958 µM).
The anti-A431 results support the SAR observation regarding the role of chlorine substitution to the indolinyl heterocycle in enhancing the antiproliferation properties relative to the unsubstituted analogs (compounds 6l and 6o are exceptions). The –I effect of the halogen atom attached to the phenyl group is also a contributory factor in the development of anti-A431 properties. Compounds with a fluorophenyl ring have lower anti-A431 proliferation values than the corresponding agents with a chlorophenyl ring. The bromophenyl-containing compound (6m) is superior to the other halogenophenyl-containing analogs. This is supported by the anti-A431 observations of compounds 6m/6i/6e (IC50 = 2.434, 4.403, 16.064 µM, respectively), 6j/6f (IC50 = 4.083, 4.764 µM, respectively), 6k/6g (IC50 = 2.966, 6.167 µM, respectively) and 6l/6h (IC50 = 3.694, 6.042 µM, respectively). The SARs are comparable to those previously discussed for anti-MCF7 and anti-HCT116 properties.
Some of the synthesized spiro-3-indolin-2-ones show anti-PaCa-2 properties with efficacy higher than that of the reference standard used (sunitinib, a drug applicable for pancreatic cancer treatment). Compounds 6j and 6k are the most promising agents against PaCa-2 (IC50 = 8.830 µM for both 6j and 6k; compared to IC50 = 16.91 µM for sunitinib). Compounds 6f,i,l,m also show promising anti-PaCa-2 properties (IC50 = 9.043‒12.500 µM).
SARs deduced from the anti-PaCa-2 properties indicate mainly the same parameters/controlling factors revealed by the previously mentioned cell lines. The chloro-substituted indolyl-containing heterocycles show higher anti-PaCa-2 efficacies than the unsubstituted analogs (compounds 6l and 6o are exceptions). The chlorophenyl-containing compounds have more enhanced anti-PaCa-2 properties than the fluorophenyl-containing analogs as shown in pairs 6i/6e (IC50 = 9.043, 39.894 µM, respectively), 6j/6f (IC50 = 8.830, 11.702 µM, respectively), 6k/6g (IC50 = 8.830, 28.404 µM, respectively) and 6l/6h (IC50 = 11.915, 14.043 µM, respectively).
The safety of the synthesized agents against non-cancer/normal RPE1 (retinal pigment epithelium) cell line was assessed (Table 1, Supplementary Fig. S52). The SI (selectivity index) for the tested compounds against RPE1 cell line confirms the safety towards non-cancer cells. Compound 6m (the most promising analog synthesized against MCF7, HCT116 and A431 cell lines) shows high SI values (SI = 4.1‒6.1). Compound 6k (potent agent against PaCa2) reveals considerable SI value (SI = 1.7). Compound 6d and 6h which are also of high anti-PaCa2 show remarkable SI values (SI = > 3.8, > 3.6, respectively).
Flow cytometry (FC) is a reliable technique for evaluation of cancer cell progression72,73,74. Illumination of the cells by lasers can identify the stained propidium iodide (PI) DNA cell content in stoichiometric population value. This is a commonly accessible methodology for studying cancer cell antiproliferation and cell cycle phase suppression75. Compounds 6l and 6m which are potent analogs against MCF7 (IC50 = 3.986, 3.597 µM) were considered for cell cycle studies by the standard PI-FC technique76 utilizing the IC50 values observed through MTT assay (Table 1, Supplementary Fig. S48).
It is noteworthy that the percentage (%) of DNA content for compound 6l was higher in G1 phase than the control experiment (% DNA = 66.13, 57.12 for compound 6l and control experiment at G1 phase, respectively). This is conclusive evidence for the ability of compound 6l to suppress the tested cell line (MCF7) by arresting the cell cycle progress at G1 phase. Meanwhile, accumulation of % DNA content was observed by compound 6m at both G1 and S phases relative to the control experiment (% DNA = 62.51, 35.11; 57.12, 29.61 for compound 6m and control experiment at G1 and S phases, respectively). This is an indication for the ability of compound 6m to affect the MCF7 cell cycle progression due to its antiproliferation properties by arresting it at G1/S phases. Decrease of G2/M phase by both the tested compounds relative to the control experiment also supports the ability of the agents for suppression cell cycle progress due to their antiproliferation properties (% DNA = 10.13, 2.38, 13.27 for compounds 6l, 6m and control experiment at G2/M phase, respectively) (Table 2, Figs. 6 and 7).
Cell cycle analysis of compounds 6l, 6m and control experiment for MCF7 (breast cancer cell line).
% DNA content of compounds 6l, 6m and control experiment for MCF7 (breast cancer cell line) at G0-G1, S and G2/M phases through PI-FC cell cycle studies.
It is also notable that both the tested compounds increase the apoptosis of the tested cancer cells77. Moreover, the total amount of apoptosis for the tested cell line is higher for compound 6m than that for 6l (total number of apoptosis = 38.41, 42.55 for compounds 6l and 6m, respectively). The late stage apoptosis of cells was observed in higher amount for compound 6l than for 6m (late stage apoptosis = 21.01, 13.71 for compounds 6l and 6m, respectively). On the other hand, necrosis observed for compound 6m is relatively higher than that of compound 6l (necrosis = 3.01, 3.96 for compounds 6l and 6m, respectively). In conclusion, it can be stated that both the tested compounds 6l and 6m are apoptosis and necrosis forming to the tested cell line due to their antiproliferation properties with compound 6m seeming more effective than 6l. These observations agree with the antiproliferation properties observed through MTT assay (Table 3, Fig. 8).
% Apoptosis and necrosis of compounds 6l, 6m and control experiment for MCF7 (breast cancer cell line).
EGFR (epidermal growth factor receptor) is a transmembrane protein tyrosine kinase involved in proliferation and differentiation in human cells (either normal or malignant). Increased EGFR activity due to overexpression is involved in many cancer types including non-small cell lung, breast, head and neck cancers. Therefore, agents targeting EGFR can serve as anticancer therapeutics with minimal off-target side effects78,79,80.
VEGFR (vascular endothelial growth factor receptor) is a cell surface tyrosine kinase receptor. Many members of the VEGFR family have been identified, indicating VEGFR-2 as the most important one. VEGFR-2 has attracted a lot of attention due to its important role against tumor-associated angiogenesis. Angiogenesis is the formation of new blood capillaries from pre-existing blood vessels. This is an essential process for many cellular functions such as proliferation, migration, and survival necessary for embryonic and adult development. Abnormal angiogenesis is associated with many diseases (such as inflammation, rheumatoid arthritis and cancer). This is the reason inhibition of VEGFR is a compelling approach to starve tumor cells and arrest solid tumor proliferation and metastasis81,82,83,84. Many small molecules have been discovered to possess VEGFR-2 inhibitory properties, including sunitinib81 (which is an indolyl scaffold with structural resemblance to the targeted synthesized agents).
The synthesized agents were considered for inhibition of EGFR and VEGFR-2 based on their solid tumor proliferation properties and chemical structural resemblance to sunitinib. The western blot technique was applied utilizing the IC50 observed for each respective agent synthesized during MTT assay85,86. Consideration of multi-targeted inhibitory properties investigation is based in the fact that cancer initiation and proliferation sometimes utilizes many receptors or signaling pathways. Clinical effectiveness reveals that single-target drugs usually suffer from cancer cell resistance due to heterogeneity of tumor cells. This is why multi-targeted agents are preferable over single-target or multi-component drug cocktails87,88.
The results for most of the synthesized agents (Table 4, Supplementary Fig. S53) reveal promising inhibitory properties against both enzymes utilized (EGFR and VEGFR-2). Compounds 6c (R = Ph, R′ = Et, R″ = H) and 6n (R = 3,4-(H3CO)2C6H3, R′ = Me, R″ = H) are the most effective of all the agents tested against EGFR, with high inhibitory properties (inhibition of EGFR = 69.6%). Compound 6f (R = 4-FC6H4, R′ = Me, R″ = Cl) and 6i (R = 4-ClC6H4, R′ = Me, R″ = H) also reveal comparable efficacies (% inhibition of EGFR = 69.3, 68.7 for 6f and 6i, respectively). SARs based on the inhibitory properties of the synthesized agents on EGFR show that the chloroindolyl-containing compounds are less potent than the unsubstituted analogs (compounds 6f and 6h are exceptions).
Compounds 6h (R = 4-FC6H4, R′ = Et, R″ = Cl) and 6k (R = 4-ClC6H4, R′ = Et, R″ = H) are the most effective agents against VEGFR-2 showing promising inhibitory properties (inhibition of VEGFR-2 = 66.2%). Compound 6e (R = 4-FC6H4, R′ = Me, R″ = H) also has comparable efficacy (inhibition of VEGFR-2 = 64.5%). SARs based on the observed inhibition of VEGFR-2 supports the observation that the fluorophenyl-containing spiro-3-indolin-2-ones have higher efficacies than unsubstituted phenyl-containing analogs [compound 6c is an exception with nearly the same efficacy to that of 6g (% inhibition of VEGFR-2 = 63.8, 63.7 for 6c and 6g, respectively)] as shown by pairs 6a/6e (% inhibition of VEGFR-2 = 60.9, 64.5, respectively), 6b/6f (% inhibition of VEGFR-2 = 64.2, 64.7, respectively) and 6d/6h (% inhibition of VEGFR-2 = 63.8, 66.2, respectively).
Slight variations in the enzymatic inhibitory results relative to the antiproliferations properties of the tested compounds can be rationalize by the difference in experimental techniques.
The Vero-E6 cell viral infection model/technique was undertaken to determine the anti-SARS-CoV-2 inhibitory properties of the synthesized spiro-3-indolin-2-ones 6a‒o44. Favipiravir89, Hydroxychloroquine and Chloroquine51 were considered as standard references (Table 5, Fig. 9). It is apparent from the results that many of the synthesized agents show efficacy against SARS-CoV-2 with potency higher than the standard references. Compound 6f (R = 4-FC6H4, R′ = Me, R″ = Cl) is the most promising of all the synthesized spiro-3-indolin-2-ones (IC50 = 7.666 µM) with about 3.3 and 4.8 times more potency than the standard references, chloroquine and hydroxychloroquine (IC50 = 24.98, 36.92 µM, respectively). Compound 6h (R = 4-FC6H4, R′ = Et, R″ = Cl; IC50 = 7.687 µM) reveals an efficacy close to that of 6f. Compounds 6b, 6k and 6m are also promising anti-SARS-CoV-2 active agents (IC50 = 8.431‒9.628 µM).
Dose–response curves for the synthesized agents against SARS-CoV-2.
Evident SARs from the results indicate that the chloroindolyl-containing compounds have higher efficacy against SARS-CoV-2 than the unsubstituted analogs (compounds 6d, 6l and 6o are exceptions). The ethylpiperidone-containing heterocycles (compounds 6g and 6k are exceptions) have lower potency against SARS-CoV-2 than the methyl-containing analogs as shown by pairs 6a/6c, 6b/6d, 6f/6h and 6j/6l (IC50 = 34.26/102.6, 9.628/171.3, 7.666/7.687, 27.09/31.45 µM, respectively). Additionally, the fluorophenyl-containing compounds display higher anti-SARS-CoV-2 properties than the unsubstituted phenyl-containing analogs as shown by pairs 6a/6e, 6b/6f, 6c/6g and 6d/6h (IC50 = 34.26/27.85, 9.628/7.666, 102.6/16.91, 171.3/7.687 µM, respectively). Acceptable safety indexes (SI) were observed for the synthesized agents. The chloroindolyl-containing heterocycles have higher SI than the unsubstituted indolyl-containing analogs (compounds 6b and 6o are exceptions).
Alzheimer’s disease is a progressive neurodegenerative illness and causes the majority of dementia cases in the elderly. Progression of the disease affects the areas of the brain responsible for memory, thoughts and language skills of the patient. In the advanced stage of the disease, the patient is rendered unable to meet their basic needs of life. Most of the medications available act to slow the progress of the symptoms. Restoring the level of cholinesterases [acetylcholinesterase (AChE), butyrylcholinesterase (BChE)] is one of the most important approaches in Alzheimer’s disease treatment90,91,92. Acetylcholine is a brain neurotransmitter with a major role in the maintenance of memory and consciousness93. AChE is the enzyme that hydrolyses acetylcholine. BChE can also regulate its level. Therefore, inhibition of both AChE and BChE is one of the main approaches for treating Alzheimer’s94,95. The synthesized agents in the current study were assessed for AChE and BChE inhibitory properties based on the fact that many indolyl-containing compounds inhibit cholinesterases96,97,98,99. Numerous reports have also referred to the cholinesterase inhibitory properties of spiro-indole-containing compounds25,26,27,28,100.
The results (Table 6) reveal that some of the synthesized spiro-3-indolin-2-ones have promising inhibitory properties against both AChE and BChE. However, none of the synthesized agents shows potency comparable to the standard reference used (donepezil). Compound 6g (R = 4-FC6H4, R′ = Et, R″ = H) is the most potent of the synthesized agents with the highest inhibitory properties against both AChE and BChE (IC50 = 2.46, 3.22 μM, respectively). Compound 6f (R = 4-FC6H4, R′ = Me, R″ = Cl) also exhibits promising inhibitory efficacies against both AChE and BChE (IC50 = 3.89, 3.75 μM, respectively). Additionally, compounds 6n and 6h have considerable inhibitory potencies against both AChE and BChE (IC50 = 5.40, 6.33; 6.30, 8.07 μM for 6n and 6h respectively).
It is evident that the fluorophenyl-containing compounds are more inhibitory against AChE than the unsubstituted phenyl-containing analogs as shown by pairs 6e/6a, 6f/6b, 6g/6c and 6h/6d (IC50 = 15.12/22.19, 3.89/27.65, 2.46/77.95, 6.30/41.01 μM, respectively). The same observation is also made against BChE with compound 6e is an exception (IC50 = 3.75/29.23, 3.22/65.41, 8.07/34.38 μM for 6f/6b, 6g/6c and 6h/6d, against BChE respectively).
The SI (selectivity index due to IC50 against AChE relative to the IC50 against BChE) of compound 6e is higher than that of the standard reference used (SI = 0.7, 0.8 for compound 6e and donepezil, respectively). This is due to its selective inhibitory properties against BChE relative to the AChE (IC50 = 15.12, 22.17 μM against AChE and BChE, respectively). It is also noted that the SI values of compounds 6g and 6h are similar to that of the standard reference (SI = 0.8). Compounds 6b and 6n have SI comparable to that of the standard reference (SI = 0.9 for compounds 6b and 6n).
Molecular modeling techniques are useful tools in medicinal chemical studies. Various techniques can be utilized in predicating new hits/leads, identifying the parameters necessary for bio-properties and understanding the exhibited bio-observations25,101. QSAR (quantitative structure–activity relationship) is one of the available approaches capable of generating mathematical models for connecting the bio-properties with physico-chemical (descriptor) parameters. Three main steps of QSAR modeling are; chemical structure optimization, descriptor calculation and validated modeling identification102. The current studies were undertaken by the CODESSA-Pro software25.
The robust two-descriptor QSAR model (R2 = 0.977, R2cvOO = 0.960, R2cvMO = 0.956) associates the antitumor properties of the synthesized spiro-3-indolin-2-ones with a wide range of bio-properties (IC50-observed = 3.597‒48.936 µM, IC50-predicted = 0.922‒46.734 µM) (Supplementary Tables S3–S5, Fig. S54). The maximum nucleophilic reactivity index for atom O (semi-empirical descriptor) has higher criterion (t = 21.368) and coefficient (5164.31) values than the other model’s descriptors. Due to this, the compound with high mathematical descriptor value estimates low antiproliferation properties as shown for compounds 6j and 6o (descriptor value = 0.00385, 0.01224 corresponding to estimated IC50 = 0.922, 46.734 µM, respectively). This descriptor also explains the low antiproliferation properties of methoxy group-containing compounds against MCF7 cell line relative to the other synthesized analogs. Fukui atomic nucleophilic reactivity index can be calculated by Eq. (1).103
where the \({\varepsilon }_{HOMO}\) , \({C}_{iHOMO}\) are the highest occupied molecular orbital energy and its coefficient, respectively.
The square root of partial surface area for atom C is a charge-related descriptor also with a high coefficient value = 936.267. Again, the compound with low mathematical coefficient value reveals potent antiproliferation properties against the tested cell lines. This is obvious in compounds 6a and 6l (descriptor value = 0.07704, 0.06474 corresponding to estimated IC50 = 19.024, 1.387 µM, respectively). Partial positively/negatively charged surface area can be calculated by Eq. (2).103
where \({S}_{A}\) stands either for the positively or negatively charged solvent accessible atomic surface area.
The two-descriptor QSAR model with good coefficient value (R2 = 0.834, R2cvOO = 0.709, R2cvMO = 0.742) expresses the antiproliferation properties of the synthesized agents against the HCT116 cell line. The model covers a wide range of biological properties (IC50-observed = 3.236‒28.511 µM, IC50-predicted = 2.153‒26.176 µM) (Supplementary Tables S6–S8, Fig. S55). Maximum n–n repulsion for bond C-N (t = 6.194) is a semi-empirical descriptor which contributes with a positive sign in the QSAR model. This explains the low efficacy of the agent with high mathematical descriptor value as shown by compound 6o relative to 6j (descriptor value = 167.7668, 168.4408 corresponding to estimated IC50 = 2.153, 26.176 µM for compounds 6j and 6o, respectively). The electron–electron repulsion between two different atoms can be calculated by Eq. (3).103
where A and B stand for two different atoms. \({P}_{\mu \nu }\mathrm{and} {P}_{\lambda \sigma }\) stand for the density matrix over the atomic basis \(\left\{\mu \nu \lambda \sigma \right\}\) . \(\langle \mu \nu |\lambda \sigma \rangle\) stands for the electron repulsion integrals for the atomic basis \(\left\{\mu \nu \lambda \sigma \right\}\) .
HA dependent HDCA-2 is a charge-related descriptor with a high coefficient value (30.3734). This also explains the low efficacy of the compounds possessing high mathematical descriptor values as revealed in compound 6n relative to 6k (descriptor value = 0.47269, 0.88784 corresponding to estimated IC50 = 2.688, 15.485 µM for compounds 6k and 6n, respectively). The area weighted surface charge of hydrogen bonding donor atoms HDCA2 can be calculated by Eq. (4).103
where \({S}_{D}\) stands for the solvent accessible surface area for the hydrogen bonding donor of the hydrogen atoms selected by the threshold charge. The \({q}_{D}\) is partial charge on the hydrogen bonding donor of the hydrogen atoms selected by the threshold charge. \({S}_{tot}\) is total solvent accessible molecular surface area.
A validated two-descriptor 2D-QSAR model (R2 = 0.898, R2cvOO = 0.800, R2cvMO = 0.816) describes the antiproliferation properties of the synthesized spiro-3-indolin-2-ones (Supplementary Tables S9–S11, Fig. S56). A wide range of bio-properties (IC50-observed = 2.434‒45.417 µM, IC50-predicted = 3.027‒62.035 µM) is covered by the model. FPSA2 fractional PPSA is a charge-related descriptor with high criterion (t = 9.256) and coefficient (2.06853) values. This explains the high efficacy antiproliferation properties of compound 6l over 6n (descriptor value = 0.56735, 1.14432 corresponding to estimated IC50 = 3.027, 62.035 µM, respectively). The fractional charge partial positive surface area can be calculated by Eq. (5).103
where the \(PPSA2\) stands for the total positively partial charged molecular surface area. \(TMSA\) stands for the total surface area of the molecule.
Average information content is a topological descriptor with a negative coefficient value (− 2.14585). For this reason, the synthesized agent with a high mathematical value represents a potent antiproliferation agent as shown by compounds 6a and 6h (descriptor value = 4.49045, 5.02123 corresponding to estimated IC50 = 28.534, 3.930 µM, respectively). The mean information content index can be calculated by Eq. (6).103
where \({n}_{i}\) stands for the atom number in the \({i}\) th class. The n is the total number of molecular atoms. k is the atomic layer numbers in the coordination sphere surrounding a specific atom.
The two-descriptor QSAR model describes the antiproliferation properties of the synthesized spiro-3-indolin-2-ones against PaCa-2 cell line in an accurate mode (R2 = 0.9573, R2cvOO = 0.938, R2cvMO = 0.944). The model covers a wide range of bio-properties including potent and mild efficacies (IC50-observed = 8.83‒48.404 µM, IC50-predicted = 7.488‒47.054 µM) (Supplementary Tables S12–S14, Fig. S57). Rotational entropy is a thermodynamic descriptor with a negative coefficient (− 9.48172). This explains the high antiproliferation efficacy of an agent with high a mathematical descriptor value as exhibited in compounds 6l over 6a (descriptor value = 37.155, 38.725 corresponding to estimated IC50 = 47.054, 7.824 µM for compounds 6a and 6l, respectively). The rotational entropy of a molecule can be calculated by Eq. (7).103
where \({I}_{j}\) stands for the principal moment of molecular inertia. The \(\sigma\) stands for molecular symmetry number with, \(h and k\) standing for Planck’s and Boltzmann’s constants. \(T\) is the absolute temperature (K).
Maximum population of an electronic atomic orbital is a semi-empirical descriptor with a negative coefficient value (− 510.133). Therefore, a compound with a low mathematical value indicates low antiproliferation properties as shown in compound 6n over 6j (descriptor value = 1.98534, 1.93405 corresponding to estimated IC50 = 7.488, 33.729 µM for compounds 6j and 6n, respectively).
The three descriptor QSAR model optimizes the anti-SARS-CoV-2 properties of the tested compounds (R2 = 0.917, R2cvOO = 0.863, R2cvMO = 0.883). The model covers a wide range of bio-properties (IC50-observed = 7.666‒171.3 µM, IC50-predicted = 7.669‒108.928 µM), including potent, mild and weak active agents (Supplementary Tables S15–S17, Fig. S58). LUMO + 1 energy is a semi-empirical descriptor with the highest coefficient value (2.66577) of the model’s descriptors. The compound with a high mathematical descriptor value has low potency as seen in compounds 6c and 6m (descriptor value = − 0.412, − 0.605 corresponding to estimated IC50 = 69.062, 8.612 µM, respectively). The LUMO (lowest unoccupied molecular orbital) energy can be calculated by Eq. (8).103
where \({\phi }_{LUMO}\) stands for the lowest unoccupied molecular orbital. \(\widehat{F}\) stands for the Fock operator.
Maximum electrophilic reactivity index for atom O is also a semi-empirical descriptor with a negative sign coefficient value (− 120.804). This explains the weak anti-SARS-CoV-2 properties of compound 6a relative to 6b (descriptor value = 0.0117, 0.009 corresponding to estimated IC50 = 27.990, 9.632 µM, respectively). Equation (1) can calculate the descriptor value.
Minimum atomic state energy for atom N is a topological descriptor with a negative coefficient value (-7.98889). This explains the weak anti-SARS-CoV-2 activity of compound 6o relative to 6m (descriptor value = 184.7028, 184.5203 corresponding to estimated IC50 = 8.612, 108.928 µM, respectively).
The most appropriate QSAR validation technique is internal validation due to the short data set utilized. Statistical validation including, the standard division and Fisher criteria support the accuracy of the QSAR models. The comparative values of the model’s coefficient (R2) to their leave-one-out (R2cvOO) and leave-many-out (R2cvMO) coefficient values also validate the optimized QSAR models. The comparative predicted properties due to the QSAR models relative to the experimentally observed values, especially for the high potent analogs, also support the molecular models which can be considered in a future study for assigning higher potent hits/leads.
In conclusion, the targeted (E)-1″-(alkanesulfonyl)-4′-aryl-5″-arylidene-1′-methyl-dispiro[indoline-3,2′-pyrrolidine-3′,3″-piperidine]-2,4″-diones 6a‒o were regioselectively synthesized through multi-component dipolar cycloaddition reaction of 1-(alkylsulfonyl)-3,5-bis(ylidene)-piperidin-4-ones 3a‒h and azomethine ylide. Single crystal X-ray studies (6b‒d,h) confirmed the structure. Some of the synthesized 3-spiro-indolin-2-ones reveal potent antiproliferation properties against diverse human cancer cells (MCF7, HCT116, A431 and PaCa2) but are safe towards normal (RPE1) cell line. Compound 6m is the most potent agent synthesized against the tested cancer cells with comparable efficacies to those of 5-fluorouracil and sunitinib (standard references). Cell cycle studies of representative examples (6l and 6m) confirm the antiproliferation properties revealed by the MTT technique and exhibit that they are apoptosis and necrosis forming. The antiproliferative agents synthesized can be considered multi-targeted inhibitors due to their properties against EGFR and VEGFR-2. Some of the synthesized 3-spiro-indolin-2-ones show promising anti-SARS-CoV-2 properties. Compound 6f is the most potent (about 3.3 and 4.8 times the efficacy of the standard references chloroquine and hydroxychloroquine, respectively). Additionally, some of the synthesized spiro-3-indolin-2-ones reveal promising inhibitory properties against both AChE and BChE. QSAR models explained the diverse biological properties that can be considered for predicting promising hits/leads in future studies.
Melting points were determined on a capillary point apparatus (Stuart SMP3) equipped with a digital thermometer. IR spectra (KBr) were recorded on a Shimadzu FT-IR 8400S spectrophotometer. Reactions were monitored using thin layer chromatography (TLC) on 0.2 mm silica gel F254 plates (Merck) utilizing various solvents for elution. The chemical structures of the synthesized compounds were characterized by nuclear magnetic resonance spectra (1H-NMR, 13C-NMR) and determined on a Bruker NMR spectrometer (500 MHz, 125 MHz for 1H and 13C, respectively). 13C-NMR spectra are fully decoupled. Chemical shifts were reported in parts per million (ppm) using the deuterated solvent peak or tetramethylsilane as an internal standard.
A mixture of equimolar amounts of the appropriate 1-alkylsulfonyl-3,5-bis(ylidene)-piperidin-4-ones 3a‒h66 (2.5 mmol) and the corresponding isatin 4a,b with sarcosine 5 in ethanol (20 ml) was boiled under reflux for the appropriate time. The separated solid upon refluxing was collected and crystallized from a suitable solvent affording the corresponding 6a‒c,e‒g,i,m‒o. For the remaining synthesized agents, the clear reaction mixture was stored at room temperature (20‒25 °C) overnight. The separated solid was collected and crystallized from a suitable solvent affording 6d,h,j-l.
Obtained from the reaction of 3a, 4a and 5, reaction time 4 h as colorless microcrystals from n-butanol, with mp 232‒234 °C and yield 83% (1.1 g). IR: νmax/cm−1 3186, 1705, 1678, 1615, 1593. 1H-NMR (DMSO-d6) δ (ppm): 1.96 (s, 3H, NCH3), 2.25 (d, J = 12.7 Hz, 1H, upfield H of piperidinyl H2C-2″), 2.65 (s, 3H, SCH3), 3.37 (br m, 1H, upfield H of pyrrolidinyl H2C-5′), 3.54 (d, J = 14.9 Hz, 1H, upfield H of piperidinyl H2C-6″), 3.84 (t, J = 9.2 Hz, 1H, downfield H of pyrrolidinyl H2C-5′), 3.93–3.98 (m, 2H, downfield H of piperidinyl H2C-2″ + downfield H of piperidinyl H2C-6″), 4.71 (t, J = 8.7 Hz, 1H, pyrrolidinyl H-4′), 6.69 (d, J = 7.4 Hz, 1H, arom. H), 6.86 (br s, 2H, arom. H), 7.09 (br s, 1H, arom. H), 7.23–7.51 (m, 11H, 10 arom. H + olefinic CH), 10.58 (s, 1H, NH). 13C-NMR (DMSO-d6) δ (ppm): 33.6, 33.7 (NCH3, SCH3), 45.7 (pyrrolidinyl HC-4′), 46.5 (piperidinyl H2C-6″), 47.9 (piperidinyl H2C-2″), 57.3 (pyrrolidinyl H2C-5′), 61.2 [spiro-C-3′ (C-3″)], 75.3 [spiro-C-3 (C-2′)], 109.1, 120.7, 124.9, 126.8, 127.1, 128.3, 128.6, 129.1, 129.48, 129.54, 129.8, 130.1, 133.8, 137.7, 138.9, 143.6 (arom. C + olefinic C), 175.1, 195.7 (C=O). Anal. Calcd. for C30H29N3O4S (527.64): C, 68.29; H, 5.54; N, 7.96. Found: C, 68.46; H, 5.81; N, 8.30.
Obtained from the reaction of 3a, 4b and 5, reaction time 7 h as colorless microcrystals from methanol, with mp 230‒232 °C and yield 90% (1.27 g). IR: νmax/cm−1 3167, 1710, 1682, 1620, 1593. 1H-NMR (DMSO-d6) δ (ppm): 1.97 (s, 3H, NCH3), 2.29 (d, J = 12.8 Hz, 1H, upfield H of piperidinyl H2C-2″), 2.66 (s, 3H, SCH3), 3.35 (t, J = 9.0 Hz, 1H, upfield H of pyrrolidinyl H2C-5′), 3.71 (d, J = 14.9 Hz, 1H, upfield H of piperidinyl H2C-6″), 3.81 (t, J = 9.5 Hz, 1H, downfield H of pyrrolidinyl H2C-5′), 3.98 (t, J = 13.4 Hz, 2H, downfield H of piperidinyl H2C-2″ + downfield H of piperidinyl H2C-6″), 4.68 (t, J = 9.0 Hz, 1H, pyrrolidinyl H-4′), 6.69 (d, J = 8.3 Hz, 1H, arom. H), 6.78 (d, J = 2.0 Hz, 1H, arom. H), 7.15 (dd, J = 2.1, 8.3 Hz, 1H, arom. H), 7.28–7.52 (m, 11H, 10 arom. H + olefinic CH), 10.74 (s, 1H, NH). 13C-NMR (DMSO-d6) δ (ppm): 33.4, 33.8 (NCH3, SCH3), 45.9 (pyrrolidinyl HC-4′), 47.0 (piperidinyl H2C-6″), 48.3 (piperidinyl H2C-2″), 57.5 (pyrrolidinyl H2C-5′), 61.5 [spiro-C-3′ (C-3″)], 75.4 [spiro-C-3 (C-2′)], 110.6, 124.9, 126.7, 127.15, 127.19, 128.4, 128.7, 128.9, 129.7, 129.8, 130.1, 133.7, 137.5, 139.0, 142.5 (arom. C + olefinic C), 174.7, 195.6 (C=O). Anal. Calcd. for C30H28ClN3O4S (562.08): C, 64.11; H, 5.02; N, 7.48. Found: C, 64.32; H, 5.21; N, 7.63.
Obtained from the reaction of 3b, 4a and 5, reaction time 4 h as yellow microcrystals from n-butanol, with mp 232‒234 °C and yield 88% (1.19 g). IR: νmax/cm−1 3163, 1713, 1678, 1616, 1597. 1H-NMR (DMSO-d6) δ (ppm): 0.97 (t, J = 7.4 Hz, 3H, CH3CH2S), 1.97 (s, 3H, NCH3), 2.34 (d, J = 12.9 Hz, 1H, upfield H of piperidinyl H2C-2″), 2.74 (sextet, J = 7.3, 14.6 Hz, 1H, upfield H of CH3CH2S), 2.89 (sextet, J = 7.4, 14.7 Hz, 1H, downfield H of CH3CH2S), 3.33 (br s, 1H, upfield H of pyrrolidinyl H2C-5′), 3.54 (d, J = 15.0 Hz, 1H, upfield H of piperidinyl H2C-6″), 3.84 (t, J = 9.4 Hz, 1H, downfield H of pyrrolidinyl H2C-5′), 4.00–4.03 (m, 2H, downfield H of piperidinyl H2C-2″ + downfield H of piperidinyl H2C-6″), 4.71 (t, J = 9.1 Hz, 1H, pyrrolidinyl H-4′), 6.70 (d, J = 7.7 Hz, 1H, arom. H), 6.87 (br d, 2H, arom. H), 7.09–7.12 (m, 1H, arom. H), 7.22 (d, J = 6.8 Hz, 2H, arom. H), 7.28 (t, J = 7.3 Hz, 1H, arom. H), 7.35–7.41 (m, 5H, arom. H), 7.46–7.49 (m, 3H, 2 arom. H + olefinic CH), 10.58 (s, 1H, NH). 13C-NMR (DMSO-d6) δ (ppm): 7.6 (CH3CH2S), 34.3 (NCH3), 42.0 (CH2S), 46.4 (pyrrolidinyl HC-4′), 47.0 (piperidinyl H2C-6″), 48.8 (piperidinyl H2C-2″), 57.7 (pyrrolidinyl H2C-5′), 61.9 [spiro-C-3′ (C-3″)], 75.8 [spiro-C-3 (C-2′)], 109.7, 121.3, 125.5, 127.4, 127.7, 128.8, 129.2, 129.7, 130.0, 130.3, 130.4, 130.6, 134.4, 138.3, 139.3, 144.1 (arom. C + olefinic C), 175.7, 196.4 (C=O). Anal. Calcd. for C31H31N3O4S (541.67): C, 68.74; H, 5.77; N, 7.76. Found: C, 69.05; H, 5.84; N, 7.99.
Obtained from the reaction of 3b, 4b and 5, reaction time 8 h as colorless microcrystals from n-butanol, with mp 207‒209 °C and yield 85% (1.22 g). IR: νmax/cm−1 3159, 1713, 1682, 1615, 1597. 1H-NMR (DMSO-d6) δ (ppm): 0.98 (t, J = 7.4 Hz, 3H, CH3CH2S), 1.98 (s, 3H, NCH3), 2.39 (d, J = 13.0 Hz, 1H, upfield H of piperidinyl H2C-2″), 2.77 (sextet, J = 7.3, 14.2 Hz, 1H, upfield H of CH3CH2S), 2.93 (sextet, J = 7.4, 14.8 Hz, 1H, downfield H of CH3CH2S), 3.35 (t, J = 8.4 Hz, 1H, upfield H of pyrrolidinyl H2C-5′), 3.74 (d, J = 14.9 Hz, 1H, upfield H of piperidinyl H2C-6″), 3.82 (t, J = 9.4 Hz, 1H, downfield H of pyrrolidinyl H2C-5′), 4.03 (d, J = 13.0, 1H, downfield H of piperidinyl H2C-2″), 4.07 (dd, J = 2.5, 15.0 Hz, 1H, downfield H of piperidinyl H2C-6″), 4.69 (t, J = 9.1 Hz, 1H, pyrrolidinyl H-4′), 6.71 (d, J = 8.3 Hz, 1H, arom. H), 6.81 (d, J = 2.2 Hz, 1H, arom. H), 7.17 (dd, J = 2.2, 8.3 Hz, 1H, arom. H), 7.27–7.30 (m, 3H, arom. H), 7.35–7.52 (m, 8H, 7 arom. H + olefinic CH), 10.75 (s, 1H, NH). 13C-NMR (DMSO-d6) δ (ppm): 7.0 (CH3CH2S), 33.8 (NCH3), 41.2 (CH2S), 46.0 (pyrrolidinyl HC-4′), 47.0 (piperidinyl H2C-6″), 48.7 (piperidinyl H2C-2″), 57.5 (pyrrolidinyl H2C-5′), 61.7 [spiro-C-3′ (C-3″)], 75.3 [spiro-C-3 (C-2′)], 110.6, 124.9, 126.7, 127.15, 127.18, 128.3, 128.7, 128.9, 129.7, 129.8, 129.9, 130.1, 133.7, 137.6, 139.0, 142.5 (arom. C + olefinic C), 174.7, 195.8 (C=O). Anal. Calcd. for C31H30ClN3O4S (576.11): C, 64.63; H, 5.25; N, 7.29. Found: C, 64.49; H, 5.37; N, 7.45.
Obtained from the reaction of 3c, 4a and 5, reaction time 5 h as colorless microcrystals from n-butanol, with mp 217‒219 °C and yield 82% (1.15 g). IR: νmax/cm−1 3186, 1701, 1620, 1601. 1H-NMR (DMSO-d6) δ (ppm): 1.95 (s, 3H, NCH3), 2.31 (d, J = 12.7 Hz, 1H, upfield H of piperidinyl H2C-2″), 2.69 (s, 3H, SCH3), 3.35 (m, 1H, upfield H of pyrrolidinyl H2C-5′), 3.51 (d, J = 15.0 Hz, 1H, upfield H of piperidinyl H2C-6″), 3.77 (t, J = 9.3 Hz, 1H, downfield H of pyrrolidinyl H2C-5′), 3.93 (t, J = 13.4 Hz, 2H, downfield H of piperidinyl H2C-2″ + downfield H of piperidinyl H2C-6″), 4.69 (t, J = 9.0 Hz, 1H, pyrrolidinyl H-4′), 6.70 (d, J = 7.7 Hz, 1H, arom. H), 6.84–6.86 (m, 2H, arom. H), 7.09 (quintet, J = 2.4, 8.1 Hz, 1H, arom. H), 7.19 (t, J = 8.6 Hz, 2H, arom. H), 7.25 (t, J = 8.7 Hz, 2H, arom. H), 7.32–7.34 (m, 2H, arom. H), 7.51–7.53 (m, 3H, 2 arom. H + olefinic CH), 10.61 (s, 1H, NH). 13C-NMR (DMSO-d6) δ (ppm): 33.6 (NCH3, SCH3), 45.0 (pyrrolidinyl HC-4′), 46.5 (piperidinyl H2C-6″), 47.8 (piperidinyl H2C-2″), 57.7 (pyrrolidinyl H2C-5′), 61.0 [spiro-C-3′ (C-3″)], 75.5 [spiro-C-3 (C-2′)], 109.1, 115.0, 115.7, 115.8, 120.7, 124.8, 126.8, 129.2, 129.4, 130.41, 130.43, 131.67, 131.74, 132.5, 132.6, 133.89, 133.91, 137.7, 143.6, 160.3, 161.4, 162.2, 163.4 (arom. C + olefinic C), 175.1, 195.6 (C=O). Anal. Calcd. for C30H27F2N3O4S (563.62): C, 63.93; H, 4.83; N, 7.46. Found: C, 64.16; H, 5.01; N, 7.66.
Obtained from the reaction of 3c, 4b and 5, reaction time 4 h as colorless microcrystals from n-butanol, with mp 212‒214 °C and yield 74% (1.10 g). IR: νmax/cm−1 3067, 1728, 1686, 1597, 1582. 1H-NMR (DMSO-d6) δ (ppm): 1.97 (s, 3H, NCH3), 2.34 (d, J = 12.8 Hz, 1H, upfield H of piperidinyl H2C-2″), 2.71 (s, 3H, SCH3), 3.37 (t, J = 8.4 Hz, 1H, upfield H of pyrrolidinyl H2C-5′), 3.68 (d, J = 14.9 Hz, 1H, upfield H of piperidinyl H2C-6″), 3.74 (t, J = 9.4 Hz, 1H, downfield H of pyrrolidinyl H2C-5′), 3.92 (d, J = 12.7 Hz, 1H, downfield H of piperidinyl H2C-2″), 4.00 (d, J = 15.0 Hz, 1H, downfield H of piperidinyl H2C-6″), 4.66 (t, J = 9.0 Hz, 1H, pyrrolidinyl H-4′), 6.70 (d, J = 8.3 Hz, 1H, arom. H), 6.77 (d, J = 1.9 Hz, 1H, arom. H), 7.16 (dd, J = 2.0, 8.4 Hz, 1H, arom. H), 7.20 (t, J = 8.7 Hz, 2H, arom. H), 7.29 (t, J = 8.8 Hz, 2H, arom. H), 7.38–7.40 (m, 2H, arom. H), 7.51–7.53 (m, 3H, 2 arom. H + olefinic CH), 10.78 (s, 1H, NH). 13C-NMR (DMSO-d6) δ (ppm): 33.4, 33.8 (NCH3, SCH3), 45.1 (pyrrolidinyl HC-4′), 47.0 (piperidinyl H2C-6″), 48.1 (piperidinyl H2C-2″), 57.9 (pyrrolidinyl H2C-5′), 61.3 [spiro-C-3′ (C-3″)], 75.5 [spiro-C-3 (C-2′)], 110.6, 115.0, 115.2, 115.7, 115.9, 124.8, 126.7, 127.0, 129.0, 129.5, 130.2, 131.73, 131.79, 132.62, 132.69, 133.7, 137.8, 142.5, 160.3, 161.5, 162.2, 163.5 (arom. C + olefinic C), 174.8, 195.5 (C=O). Anal. Calcd. for C30H26ClF2N3O4S (598.06): C, 60.25; H, 4.38; N, 7.03. Found: C, 60.44; H, 4.54; N, 7.17.
Obtained from the reaction of 3d, 4a and 5, reaction time 4 h as yellow microcrystals from n-butanol, with mp 220‒222 °C and yield 76% (1.09 g). IR: νmax/cm−1 3182, 1713, 1682, 1620, 1601. 1H-NMR (DMSO-d6) δ (ppm): 1.00 (t, J = 7.4 Hz, 3H, CH3CH2S), 1.96 (s, 3H, NCH3), 2.39 (d, J = 12.9 Hz, 1H, upfield H of piperidinyl H2C-2″), 2.79 (sextet, J = 7.3, 14.6 Hz, 1H, upfield H of CH3CH2S), 2.92 (sextet, J = 7.4, 14.8 Hz, 1H, downfield H of CH3CH2S), 3.35 (t, J = 8.4 Hz, 1H, upfield H of pyrrolidinyl H2C-5′), 3.52 (d, J = 15.0 Hz, 1H, upfield H of piperidinyl H2C-6″), 3.77 (t, J = 9.4 Hz, 1H, downfield H of pyrrolidinyl H2C-5′), 3.96 (d, J = 12.8 Hz, 1H, downfield H of piperidinyl H2C-2″), 4.01 (dd, J = 2.2, 15.0 Hz, 1H, downfield H of piperidinyl H2C-6″), 4.69 (t, J = 9.0 Hz, 1H, pyrrolidinyl H-4′), 6.71 (d, J = 7.7 Hz, 1H, arom. H), 6.85–6.87 (m, 2H, arom. H), 7.09–7.12 (m, 1H, arom. H), 7.19 (t, J = 8.8 Hz, 2H, arom. H), 7.25 (t, J = 8.8 Hz, 2H, arom. H), 7.30–7.33 (m, 2H, arom. H), 7.50–7.53 (m, 3H, 2 arom. H + olefinic CH), 10.61 (s, 1H, NH). 13C-NMR (DMSO-d6) δ (ppm): 7.0 (CH3CH2S), 33.7 (NCH3), 41.3 (CH2S), 45.1 (pyrrolidinyl HC-4′), 46.4 (piperidinyl H2C-6″), 48.1 (piperidinyl H2C-2″), 57.6 (pyrrolidinyl H2C-5′), 61.2 [spiro-C-3′ (C-3″)], 75.4 [spiro-C-3 (C-2′)], 109.2, 115.0, 115.1, 115.7, 115.8, 120.7, 124.8, 126.8, 129.2, 129.60, 129.62, 130.4, 131.69, 131.76, 132.5, 132.6, 133.94, 133.96, 137.6, 143.6, 160.3, 161.4, 162.2, 163.4 (arom. C + olefinic C), 175.1, 195.7 (C=O). Anal. Calcd. for C31H29F2N3O4S (577.65): C, 64.46; H, 5.06; N, 7.27. Found: C, 64.68; H, 4.86; N, 6.96.
Obtained from the reaction of 3d, 4b and 5, reaction time 6h as colorless microcrystals from ethanol, with mp 136‒138 °C and yield 78% (1.20 g). IR: νmax/cm−1 3341, 1694, 1620, 1601, 1582. 1H-NMR (DMSO-d6) δ (ppm): 1.07 (t, J = 7.0 Hz, 3H, CH3CH2S), 1.98 (s, 3H, NCH3), 2.45 (d, J = 12.9 Hz, 1H, upfield H of piperidinyl H2C-2″), 2.82 (sextet, J = 7.3, 14.6 Hz, 1H, upfield H of CH3CH2S), 2.96 (sextet, J = 7.4, 14.8 Hz, 1H, downfield H of CH3CH2S), 3.38 (t, J = 8.5 Hz, 1H, upfield H of pyrrolidinyl H2C-5′), 3.71 (d, J = 15.0 Hz, 1H, upfield H of piperidinyl H2C-6″), 3.76 (t, J = 9.4 Hz, 1H, downfield H of pyrrolidinyl H2C-5′), 3.99 (d, J = 12.9 Hz, 1H, downfield H of piperidinyl H2C-2″), 4.08 (dd, J = 2.2, 14.9 Hz, 1H, downfield H of piperidinyl H2C-6″), 4.68 (t, J = 9.0 Hz, 1H, pyrrolidinyl H-4′), 6.72 (d, J = 8.3 Hz, 1H, arom. H), 6.80 (d, J = 2.1 Hz, 1H, arom. H), 7.17 (dd, J = 2.3, 8.2 Hz, 1H, arom. H), 7.19 (t, J = 8.5 Hz, 2H, arom. H). 7.29 (t, J = 8.8 Hz, 2H, arom. H), 7.37–7.40 (m, 2H, arom. H), 7.52–7.55 (m, 3H, arom. H + olefinic CH), 10.78 (s, 1H, NH). 13C-NMR (DMSO-d6) δ (ppm): 7.0 (CH3CH2S), 33.9 (NCH3), 41.2 (CH2S), 45.3 (pyrrolidinyl HC-4′), 47.0 (piperidinyl H2C-6″), 48.6 (piperidinyl H2C-2″), 57.9 (pyrrolidinyl H2C-5′), 61.6 [spiro-C-3′ (C-3″)], 75.5 [spiro-C-3 (C-2′)], 110.7, 115.0, 115.2, 115.8, 116.0, 125.0, 126.8, 127.1, 129.0, 129.77, 129.79, 130.28, 130.30, 131.81, 131.87, 132.60, 132.67, 133.8, 137.8, 142.6, 160.4, 161.6, 162.3, 163.6 (arom. C + olefinic C), 174.8, 195.7 (C=O). Anal. Calcd. for C31H28ClF2N3O4S (612.09): C, 60.83; H, 4.61; N, 6.87. Found: C, 61.01; H, 4.75; N, 6.96.
Obtained from the reaction of 3e, 4a and 5, reaction time 4 h as colorless microcrystals from ethanol, with mp 221‒223 °C and yield 87% (1.30 g). IR: νmax/cm−1 3387, 1697, 1618, 1605. 1H-NMR (DMSO-d6) δ (ppm): 1.95 (s, 3H, NCH3), 2.34 (d, J = 12.7 Hz, 1H, upfield H of piperidinyl H2C-2″), 2.70 (s, 3H, SCH3), 3.34 (t, J = 8.1 Hz, 1H, upfield H of pyrrolidinyl H2C-5′), 3.51 (d, J = 15.1 Hz, 1H, upfield H of piperidinyl H2C-6″), 3.77 (t, J = 9.3 Hz, 1H, downfield H of pyrrolidinyl H2C-5′), 3.91–3.95 (m, 2H, downfield H of piperidinyl H2C-2″ + downfield H of piperidinyl H2C-6″), 4.68 (t, J = 9.0 Hz, 1H, pyrrolidinyl H-4′), 6.70 (d, J = 7.7 Hz, 1H, arom. H), 6.84–6.85 (m, 2H, arom. H), 7.08–7.11 (m, 1H, arom. H), 7.28 (d, J = 8.4 Hz, 2H, arom. H), 7.42 (d, J = 8.3 Hz, 2H, arom. H), 7.46–7.51 (m, 5H, 4 arom. H + olefinic CH), 10.62 (s, 1H, NH). 13C-NMR (DMSO-d6) δ (ppm): 33.7 (NCH3, SCH3), 45.1 (pyrrolidinyl HC-4′), 46.5 (piperidinyl H2C-6″), 47.7 (piperidinyl H2C-2″), 57.5 (pyrrolidinyl H2C-5′), 61.1 [spiro-C-3′ (C-3″)], 75.4 [spiro-C-3 (C-2′)], 109.2, 120.7, 124.7, 126.9, 128.3, 128.7, 129.2, 130.1, 131.7, 131.8, 132.7, 134.3, 136.8, 137.5, 143.6 (arom. C + olefinic C), 175.1, 195.5 (C=O). Anal. Calcd. for C30H27Cl2N3O4S (596.52): C, 60.41; H, 4.56; N, 7.04. Found: C, 60.16; H, 4.30; N, 6.90.
Obtained from the reaction of 3e, 4b and 5, reaction time 8 h as colorless microcrystals from n-butanol, with mp 219‒221 °C and yield 74% (1.17 g). IR: νmax/cm−1 3136, 3098, 1730, 1694, 1612. 1H-NMR (DMSO-d6) δ (ppm): 1.97 (s, 3H, NCH3), 2.40 (d, J = 12.8 Hz, 1H, upfield H of piperidinyl H2C-2″), 2.73 (s, 3H, SCH3), 3.38 (t, J = 8.8 Hz, 1H, upfield H of pyrrolidinyl H2C-5′), 3.69 (d, J = 15.0 Hz, 1H, upfield H of piperidinyl H2C-6″), 3.75 (t, J = 9.4 Hz, 1H, downfield H of pyrrolidinyl H2C-5′), 3.94 (d, J = 12.7 Hz, 1H, downfield H of piperidinyl H2C-2″), 4.01 (d, J = 15.1 Hz, 1H, downfield H of piperidinyl H2C-6″), 4.66 (t, J = 9.0 Hz, 1H, pyrrolidinyl H-4′), 6.71 (d, J = 8.3 Hz, 1H, arom. H), 6.77 (d, J = 1.7 Hz, 1H, arom. H), 7.16 (dd, J = 2.0, 8.3 Hz, 1H, arom. H), 7.34 (d, J = 8.5 Hz, 2H, arom. H), 7.42 (d, J = 8.3 Hz, 2H, arom. H), 7.50–7.52 (m, 5H, 4 arom. H + olefinic CH), 10.79 (s, 1H, NH). 13C-NMR (DMSO-d6) δ (ppm): 33.6, 33.8 (NCH3, SCH3), 45.2 (pyrrolidinyl HC-4′), 47.0 (piperidinyl H2C-6″), 48.0 (piperidinyl H2C-2″), 57.8 (pyrrolidinyl H2C-5′), 61.4 [spiro-C-3′ (C-3″)], 75.5 [spiro-C-3 (C-2′)], 110.7, 124.9, 126.7, 127.0, 128.3, 128.8, 129.0, 130.3, 131.8, 131.9, 132.5, 134.5, 136.6, 137.6, 142.6 (arom. C + olefinic C), 174.7, 195.3 (C=O). Anal. Calcd. for C30H26Cl3N3O4S (630.97): C, 57.11; H, 4.15; N, 6.66. Found: C, 57.21; H, 3.96; N, 6.88.
Obtained from the reaction of 3f, 4a and 5, reaction time 5 h as colorless microcrystals from ethanol, with mp 136‒138 °C and yield 86% (1.31 g). IR: νmax/cm−1 3379, 1697, 1619, 1601. 1H-NMR (DMSO-d6) δ (ppm): 1.01 (t, J = 7.4 Hz, 3H, CH3CH2S), 1.95 (s, 3H, NCH3), 2.42 (d, J = 12.9 Hz, 1H, upfield H of piperidinyl H2C-2″), 2.78 (sextet, J = 7.3, 14.5 Hz, 1H, upfield H of CH3CH2S), 2.93 (sextet, J = 7.4, 14.7 Hz, 1H, downfield H of CH3CH2S), 3.34 (t, J = 8.3 Hz, 1H, upfield H of pyrrolidinyl H2C-5′), 3.51 (d, J = 15.1 Hz, 1H, upfield H of piperidinyl H2C-6″), 3.76 (t, J = 9.3 Hz, 1H, downfield H of pyrrolidinyl H2C-5′), 3.96 (d, J = 12.8 Hz, 1H, downfield H of piperidinyl H2C-2″), 4.00 (dd, J = 1.7, 15.1 Hz, 1H, downfield H of piperidinyl H2C-6″), 4.68 (t, J = 9.0 Hz, 1H, pyrrolidinyl H-4′), 6.70 (d, J = 7.7 Hz, 1H, arom. H), 6.85 (s, 1H, arom. H), 6.86 (s, 1H, arom. H), 7.09–7.12 (m, 1H, arom. H), 7.25 (d, J = 8.5 Hz, 2H, arom. H), 7.42 (d, J = 8.4 Hz, 2H, arom. H), 7.46–7.51 (m, 5H, 4 arom. H + olefinic CH), 10.61 (s, 1H, NH). 13C-NMR (DMSO-d6) δ (ppm): 7.0 (CH3CH2S), 33.7 (NCH3), 41.4 (CH2S), 45.2 (pyrrolidinyl HC-4′), 46.4 (piperidinyl H2C-6″), 48.0 (piperidinyl H2C-2″), 57.4 (pyrrolidinyl H2C-5′), 61.3 [spiro-C-3′ (C-3″)], 75.3 [spiro-C-3 (C-2′)], 109.3, 120.7, 124.7, 126.9, 128.4, 128.7, 129.2, 130.4, 131.7, 132.7, 134.2, 136.8, 137.4, 143.6 (arom. C + olefinic C), 175.1, 195.6 (C=O). Anal. Calcd. for C31H29Cl2N3O4S (610.55): C, 60.98; H, 4.79; N, 6.88. Found: C, 60.81; H, 4.63; N, 6.75.
Obtained from the reaction of 3f, 4b and 5, reaction time 6h as colorless microcrystals from ethanol, with mp 133‒136 °C and yield 75% (1.20 g). IR: νmax/cm−1 3356, 1694, 1620, 1597. 1H-NMR (DMSO-d6) δ (ppm): 1.01 (t, J = 7.4 Hz, 3H, CH3CH2S), 1.95 (s, 3H, NCH3), 2.42 (d, J = 12.9 Hz, 1H, upfield H of piperidinyl H2C-2″), 2.79 (sextet, J = 7.2, 14.2 Hz, 1H, upfield H of CH3CH2S), 2.93 (sextet, J = 7.4, 14.7 Hz, 1H, downfield H of CH3CH2S), 3.34 (t, J = 8.3 Hz, 1H, upfield H of pyrrolidinyl H2C-5′), 3.51 (d, J = 15.1 Hz, 1H, upfield H of piperidinyl H2C-6″), 3.76 (t, J = 9.3 Hz, 1H, downfield H of pyrrolidinyl H2C-5′), 3.96 (d, J = 12.8 Hz, 1H, downfield H of piperidinyl H2C-2″), 4.00 (dd, J = 1.7, 15.1 Hz, 1H, downfield H of piperidinyl H2C-6″), 4.68 (t, J = 9.0 Hz, 1H, pyrrolidinyl H-4′), 6.70 (d, J = 7.7 Hz, 1H, arom. H), 6.85 (s, 1H, arom. H), 6.86 (s, 1H, arom. H), 7.09–7.12 (m, 1H, arom. H), 7.25 (d, J = 8.5 Hz, 2H, arom. H), 7.42 (d, J = 8.4 Hz, 2H, arom. H), 7.46–7.51 (m, 4H, 3 arom. H + olefinic CH), 10.61 (s, 1H, NH). 13C-NMR (DMSO-d6) δ (ppm): 6.9 (CH3CH2S), 33.8 (NCH3), 41.2 (CH2S), 45.4 (pyrrolidinyl HC-4′), 46.9 (piperidinyl H2C-6″), 48.4 (piperidinyl H2C-2″), 57.7 (pyrrolidinyl H2C-5′), 61.6 [spiro-C-3′ (C-3″)], 75.5 [spiro-C-3 (C-2′)], 110.8, 124.9, 126.7, 127.0, 128.3, 128.8, 129.1, 130.5, 131.81, 131.84, 131.86, 132.5, 134.5, 136.6, 137.5, 142.6 (arom. C + olefinic C), 174.8, 195.5 (C=O). Anal. Calcd. for C31H28Cl3N3O4S (644.99): C, 57.73; H, 4.38; N, 6.51. Found: C, 57.52; H, 4.28; N, 6.57.
Obtained from the reaction of 3g, 4a and 5, reaction time 12 h as pale yellow microcrystals from n-butanol, with mp 224‒226 °C and yield 70% (1.20 g). IR: νmax/cm−1 3306, 1717, 1674, 1610. 1H-NMR (DMSO-d6) δ (ppm): 1.95 (s, 3H, NCH3), 2.35 (d, J = 12.7 Hz, 1H, upfield H of piperidinyl H2C-2″), 2.70 (s, 3H, SCH3), 3.35 (t, J = 6.5 Hz, 1H, upfield H of pyrrolidinyl H2C-5′), 3.50 (d, J = 15.1 Hz, 1H, upfield H of piperidinyl H2C-6″), 3.76 (t, J = 9.3 Hz, 1H, downfield H of pyrrolidinyl H2C-5′), 3.91–3.94 (m, 2H, downfield H of piperidinyl H2C-2″ + downfield H of piperidinyl H2C-6″), 4.67 (t, J = 8.9 Hz, 1H, pyrrolidinyl H-4′), 6.70 (d, J = 7.7 Hz, 1H, arom. H), 6.84–6.85 (br d, 2H, arom. H), 7.08–7.11 (m, 1H, arom. H), 7.20 (d, J = 8.3 Hz, 2H, arom. H), 7.44–7.73 (m, 7H, 6 arom. H + olefinic CH), 10.62 (s, 1H, NH). 13C-NMR (DMSO-d6) δ (ppm): 33.7, 36.0 (NCH3, SCH3), 45.1 (pyrrolidinyl HC-4′), 46.5 (piperidinyl H2C-6″), 47.7 (piperidinyl H2C-2″), 57.4 (pyrrolidinyl H2C-5′), 61.1 [spiro-C-3′ (C-3″)], 75.4 [spiro-C-3 (C-2′)], 109.2, 120.3, 120.6, 123.1, 123.3, 124.7, 126.8, 129.2, 130.2, 131.2, 131.6, 131.8, 132.0, 132.1, 132.4, 133.0, 133.1, 135.6, 137.2, 137.5, 143.6 (arom. C + olefinic C), 175.1, 195.5 (C=O). Anal. Calcd. for C30H27Br2N3O4S (685.43): C, 52.57; H, 3.97; N, 6.13. Found: C, 52.75; H, 4.11; N, 6.30.
Obtained from the reaction of 3h, 4a and 5, reaction time 10 h as yellow microcrystals from n-butanol, with mp 204‒206 °C and yield 93% (1.50 g). IR: νmax/cm−1 3345, 1717, 1663, 1582. 1H-NMR (DMSO-d6) δ (ppm): 1.95 (s, 3H, NCH3), 2.27 (d, J = 12.8 Hz, 1H, upfield H of piperidinyl H2C-2″), 2.69 (s, 3H, SCH3), 3.33 (t, J = 8.4 Hz, 1H, upfield H of pyrrolidinyl H2C-5′), 3.50 (d, J = 14.9 Hz, 1H, upfield H of piperidinyl H2C-6″), 3.75–3.78 (m, 13H, 4 OCH3 + downfield H of pyrrolidinyl H2C-5′), 3.97 (dd, J = 1.8, 15.0 Hz, 1H, downfield H of piperidinyl H2C-6″), 4.01 (d, J = 12.8 Hz, 1H, downfield H of piperidinyl H2C-2″), 4.60 (t, J = 9.0 Hz, 1H, pyrrolidinyl H-4′), 6.70 (d, J = 7.7 Hz, 1H, arom. H), 6.77 (d, J = 7.5 Hz, 1H, arom. H), 6.82–6.86 (m, 2H, arom. H), 6.90–7.00 (m, 4H, arom. H), 7.09–7.51 (m, 3H, 2 arom. H + olefinic CH), 10.57 (s, 1H, NH). 13C-NMR (DMSO-d6) δ (ppm): 33.8 (NCH3, SCH3), 45.5 (pyrrolidinyl HC-4′), 46.5 (piperidinyl H2C-6″), 47.6 (piperidinyl H2C-2″), 55.3, 55.4 (OCH3), 57.9 (pyrrolidinyl H2C-5′), 61.0 [spiro-C-3′ (C-3″)], 75.5 [spiro-C-3 (C-2′)], 109.0, 111.4, 111.5, 113.6, 114.3, 120.6, 122.0, 123.4, 125.1, 126.6, 126.7, 127.6, 129.0, 130.1, 139.1, 143.5, 147.8, 148.4, 150.1 (arom. C + olefinic C), 175.3, 195.6 (C=O). Anal. Calcd. for C34H37N3O8S (647.74): C, 63.05; H, 5.76; N, 6.49. Found: C, 62.74; H, 5.94; N, 6.22.
Obtained from the reaction of 3h, 4b and 5, reaction time 12 h as yellow microcrystals from n-butanol, with mp 201‒203 °C and yield 74% (1.27 g). IR: νmax/cm−1 3345, 1717, 1663, 1582. 1H-NMR (DMSO-d6) δ (ppm): 1.96 (s, 3H, NCH3), 2.27 (d, J = 12.9 Hz, 1H, upfield H of piperidinyl H2C-2″), 2.69 (s, 3H, SCH3), 3.33 (t, J = 8.4 Hz, 1H, upfield H of pyrrolidinyl H2C-5′), 3.50 (d, J = 14.9 Hz, 1H, upfield H of piperidinyl H2C-6″), 3.75–3.78 (m, 13H, 4 OCH3 + downfield H of pyrrolidinyl H2C-5′), 3.96–4.03 (m, 2H, downfield H of piperidinyl H2C-2″ + downfield H of piperidinyl H2C-6″), 4.60 (t, J = 9.0 Hz, 1H, pyrrolidinyl H-4′), 6.71 (d, J = 7.7 Hz, 1H, arom. H), 6.77 (d, J = 8.4 Hz, 1H, arom. H), 6.82–6.87 (m, 2H, arom. H), 6.90–7.00 (m, 3H, arom. H), 7.09–7.51 (m, 3H, 2 arom. H + olefinic CH), 10.58 (s, 1H, NH). 13C-NMR (DMSO-d6) δ (ppm): 33.8 (NCH3, SCH3), 45.5 (pyrrolidinyl HC-4′), 46.5 (piperidinyl H2C-6″), 47.6 (piperidinyl H2C-2″), 55.3 (OCH3), 58.0 (pyrrolidinyl H2C-5′), 61.0 [spiro-C-3′ (C-3″)], 75.5 [spiro-C-3 (C-2′)], 109.0, 111.4, 111.5, 113.6, 114.3, 120.6, 122.0, 123.4, 125.1, 126.6, 126.7, 127.6, 129.0, 130.2, 139.1, 143.5, 147.8, 148.4, 150.1 (arom. C + olefinic C), 175.3, 195.6 (C=O). Anal. Calcd. for C34H36ClN3O8S (682.19): C, 59.86; H, 5.32; N, 6.16. Found: C, 59.97; H, 5.41; N, 6.06.
All data generated or analyzed during this study are included in this published article and its Supplementary Information Files. The X-ray data have been deposited in the CSD with reference numbers CCDC 2087291, 2087292, 2087297, 2087299 and the Check-CIF files are also attached as supplementary files to this article.
Marti, C. & Carreira, E. M. Construction of spiro[pyrrolidine-3,3′-oxindoles]—Recent applications to the synthesis of oxindole alkaloids. Eur. J. Org. Chem. https://doi.org/10.1002/ejoc.200300050 (2003).
Attanasi, O. A., Campisi, L. A., De Crescentini, L., Favi, G. & Mantellini, F. Synthesis of novel symmetrical 2-oxo-spiro-[indole-3,4′-pyridines] by a reaction of oxindoles with 1,2-diaza-1,3-dienes. Org. Biomol. Chem. 13, 277–282. https://doi.org/10.1039/c4ob01959h (2015).
Sengupta, A. et al. Pseudo five component reaction towards densely functionalized spiro[indole-3,2′-pyrrole] by picric acid, an efficient syn-diastereoselective catalyst: Insight into the diastereoselection on C(sp3)–C(sp3) axial conformation. Org. Biomol. Chem. 17, 1254–1265. https://doi.org/10.1039/c8ob02849d (2019).
Article CAS PubMed Google Scholar
Huang, A., Kodanko, J. J. & Overman, L. E. Asymmetric synthesis of pyrrolidinoindolines. Application for the practical total synthesis of (–)-phenserine. J. Am. Chem. Soc. 126, 14043–14053. https://doi.org/10.1021/ja046690e (2004).
Article CAS PubMed Google Scholar
Bagul, T. D., Lakshmaiah, G., Kawabata, T. & Fuji, K. Total synthesis of spirotryprostatin B via asymmetric bitroolefination. Org. Lett. 4, 249–251. https://doi.org/10.1021/ol016999s (2002).
Article CAS PubMed Google Scholar
Shanmugam, P., Viswambharan, B., Selvakumar, K. & Madhavan, S. A facile and efficient synthesis of highly functionalized 3,3′-dispiropyrrolidine- and 3,3′-dispiropyrrolizidine bisoxindoles via [3+2] cycloaddition. Tetrahedron Lett. 49, 2611–2615. https://doi.org/10.1016/j.tetlet.2008.02.104 (2008).
Zhang, X.-C., Cao, S.-H., Wei, Y. & Shi, M. Phosphine- and nitrogen-containing Lewis base catalyzed highly regioselective and geometric selective cyclization of isatin derived electron-deficient alkenes with ethyl 2,3-butadienoate. Org. Lett. 13, 1142–1145. https://doi.org/10.1021/ol1031798 (2011).
Article ADS CAS PubMed Google Scholar
Girgis, A. S., Stawinski, J., Ismail, N. S. M. & Farag, H. Synthesis and QSAR study of novel cytotoxic spiro[3H-indole-3,2′(1′H)-pyrrolo[3,4-c]pyrrole]-2,3′,5′(1H,2′aH,4′H)-triones. Eur. J. Med. Chem. 47, 312–322. https://doi.org/10.1016/j.ejmech.2011.10.058 (2012).
Article CAS PubMed Google Scholar
Girgis, A. S. et al. Synthesis and DFT studies of an antitumor active spiro-oxindole. N. J. Chem. 39, 8017–8027. https://doi.org/10.1039/c5nj01109d (2015).
Riesco-Dominguez, A., van der Zwaluw, N., Blanco-Ania, D. & Rutjes, F. P. J. T. An enantio- and diastereoselective Mannich/Pictet–Spengler sequence to form spiro[piperidine-pyridoindoles] and application to library synthesis. Eur. J. Org. Chem. https://doi.org/10.1002/ejoc.201601508 (2017).
Kawasaki, T. et al. Synthesis of diversely functionalized hexahydropyrrolo[2,3-b]indoles using domino reactions, olefination, isomerization and Claisen rearrangement followed by reductive cyclization. J. Org. Chem. 70, 2957–2966. https://doi.org/10.1021/jo040289t (2005).
Article CAS PubMed Google Scholar
Mao, Z. & Baldwin, S. W. New spirocyclic oxindole synthesis based on a hetero Claisen rearrangement. Org. Lett. 6, 2425–2428. https://doi.org/10.1021/ol0491888 (2004).
Article CAS PubMed Google Scholar
Viswambharan, B., Selvakumar, K., Madhavan, S. & Shanmugam, P. Pyridine core activation via 1,5-electrocyclization of vinyl pyridinium ylides generated from bromo isomerized Morita-Baylis-Hillman adduct of isatin and pyridine: Synthesis of 3-spirodihydroindolizine oxindoles. Org. Lett. 12, 2108–2111. https://doi.org/10.1021/ol100591r (2010).
Article CAS PubMed Google Scholar
Lan, Y. et al. Indole methylation protects diketopiperazine configuration in the maremycin biosynthetic pathway. Sci. China Chem. 59, 1224–1228. https://doi.org/10.1007/s11426-016-0026-7 (2016).
Duan, Y. et al. Divergent biosynthesis of indole alkaloids FR900452 and spiro-maremycins. Org. Biomol. Chem. 16, 5446–5451. https://doi.org/10.1039/c8ob01181h (2018).
Article CAS PubMed Google Scholar
Tang, Y.-Q., Sattler, I., Thiericke, R., Grabley, S. & Feng, X.-Z. Maremycins C and D, new diketopiperazines, and maremycins E and F, novel polycyclic spiro-indole metabolites isolated from Streptomyces sp. Eur. J. Org. Chem. https://doi.org/10.1002/1099-0690(200101)2001:2%3c261::AID-EJOC261%3e3.0.CO;2-6 (2001).
Ding, K. et al. Structure-based design of potent non-peptide MDM2 inhibitors. J. Am. Chem. Soc. 127, 10130–10131. https://doi.org/10.1021/ja051147z (2005).
Article CAS PubMed Google Scholar
Angenot, L. New oxindole alkaloids from Strychnos usambarensis Gilg. Plant Med. Phytother. 12, 123–129 (1978).
Bassleer, R. et al. Effects of three alkaloids isolated from Strychnos usambarensis on cancer cells in culture. Planta Med. 45, 123–126 (1982).
Singh, G. S. & Desta, Z. Y. Isatins as privileged molecules in design and synthesis of spiro-fused cyclic frameworks. Chem. Rev. 112, 6104–6155. https://doi.org/10.1021/cr300135y (2012).
Article CAS PubMed Google Scholar
Thomas, N. V., Sathi, V., Deepthi, A., Leena, S. S. & Chopra, S. Engaging thieno[2,3-b]indole-2,3-dione for the efficient synthesis of spiro[indoline-3,4′-thiopyrano[2,3-b]indole] by reaction with N-substituted isatilidenes. J. Heterocyclic Chem. 58, 48–55. https://doi.org/10.1002/jhet.4147 (2021).
Pourshab, M., Asghari, S. & Mohseni, M. Synthesis and antibacterial evaluation of novel spiro[indole-pyrimidine]ones. J. Heterocyclic Chem. 55, 173–180. https://doi.org/10.1002/jhet.3021 (2018).
Sakhuja, R., Panda, S. S., Khanna, L., Khurana, S. & Jain, S. C. Design and synthesis of spiro[indole-thiazolidine]spiro[indole-pyrans] as antimicrobial agents. Bioorg. Med. Chem. Lett. 21, 5465–5469. https://doi.org/10.1016/j.bmcl.2011.06.121 (2011).
Article CAS PubMed Google Scholar
Dandia, A. et al. Efficient microwave enhanced regioselective synthesis of a series of benzimidazolyl/triazolyl spiro [indole-thiazolidinones] as potent antifungal agents and crystal structure of spiro[3H-indole-3,2′-thiazolidine]-3′(1,2,4-triazol-3-yl)-2,4′(1H)-dione. Bioorg. Med. Chem. 14, 2409–2417. https://doi.org/10.1016/j.bmc.2005.11.025 (2006).
Article CAS PubMed Google Scholar
Youssef, M. A. et al. Synthesis and molecular modeling studies of cholinesterase inhibitor dispiro[indoline-3,2′-pyrrolidine-3′,3″-pyrrolidines]. RSC Adv. 10, 21830–21838. https://doi.org/10.1039/d0ră4c (2020).
Article ADS CAS PubMed PubMed Central Google Scholar
Kia, Y. et al. Synthesis and discovery of novel piperidone-grafted mono- and bis-spirooxindole-hexahydropyrrolizines as potent cholinesterase inhibitors. Bioorg. Med. Chem. 21, 1696–1707. https://doi.org/10.1016/j.bmc.2013.01.066 (2013).
Article CAS PubMed Google Scholar
Kia, Y., Osman, H., Kumar, R. S., Basiri, A. & Murugaiyah, V. Synthesis and discovery of highly functionalized mono- and bis-spiro-pyrrolidines as potent cholinesterase enzyme inhibitors. Bioorg. Med. Chem. Lett. 24, 1815–1819. https://doi.org/10.1016/j.bmcl.2014.02.019 (2014).
Article CAS PubMed Google Scholar
Kia, Y. et al. A facile chemo-, regio- and stereoselective synthesis and cholinesterase inhibitory activity of spirooxindole–pyrrolizine–piperidine hybrids. Bioorg. Med. Chem. Lett. 23, 2979–2983. https://doi.org/10.1016/j.bmcl.2013.03.027 (2013).
Article CAS PubMed Google Scholar
Shao, C., Wang, Y. & Jin, G. Design, synthesis and biological activity of bis-sulfonyl-BODIPY probes for tumor cell imaging. Bioorg. Med. Chem. Lett. 49, 128292. https://doi.org/10.1016/j.bmcl.2021.128292 (2021).
Article CAS PubMed Google Scholar
Koutsopoulos, K. et al. Design synthesis and evaluation of novel aldose reductase inhibitors: The case of indolyl–sulfonyl–phenols. Bioorg. Med. Chem. 28, 115575. https://doi.org/10.1016/j.bmc.2020.115575 (2020).
Article CAS PubMed Google Scholar
Wan, Y., Fang, G., Chen, H., Deng, X. & Tang, Z. Sulfonamide derivatives as potential anti-cancer agents and their SARs elucidation. Eur. J. Med. Chem. 226, 113837. https://doi.org/10.1016/j.ejmech.2021.113837 (2021).
Article CAS PubMed Google Scholar
Ballatore, C., Huryn, D. M. & Smith, A. B. III. Carboxylic acid (bio)isosteres in drug design. ChemMedChem 8, 385–395. https://doi.org/10.1002/cmdc.201200585 (2013).
Article CAS PubMed PubMed Central Google Scholar
https://www.cancer.gov/about-cancer/treatment/drugs/belinostat.
https://www.drugs.com/history/beleodaq.html.
https://www.cancer.gov/about-cancer/treatment/drugs/vemurafenib.
https://www.drugs.com/history/zelboraf.html.
https://www.cancer.gov/about-cancer/treatment/drugs/dabrafenib.
https://www.drugs.com/history/tafinlar.html.
Erigür, E. C., Altuğ, C., Angeli, A. & Supuran, C. T. Design, synthesis and human carbonic anhydrase I, II, IX and XII inhibitory properties of 1,3-thiazole sulfonamides. Bioorg. Med. Chem. Lett. 59, 128581. https://doi.org/10.1016/j.bmcl.2022.128581 (2022).
Article CAS PubMed Google Scholar
Manzoor, S., Petreni, A., Raza, M. K., Supuran, C. T. & Hoda, N. Novel triazole-sulfonamide bearing pyrimidine moieties with carbonic anhydrase inhibitory action: Design, synthesis, computational and enzyme inhibition studies. Bioorg. Med. Chem. Lett. 48, 128249. https://doi.org/10.1016/j.bmcl.2021.128249 (2021).
Article CAS PubMed Google Scholar
Manasa, K. L. et al. Design and synthesis of β-carboline linked aryl sulfonyl piperazine derivatives: DNA topoisomerase II inhibition with DNA binding and apoptosis inducing ability. Bioorg. Chem. 101, 103983. https://doi.org/10.1016/j.bioorg.2020.103983 (2020).
Jarak, I. et al. Pluronic-based nanovehicles: Recent advances in anticancer therapeutic applications. Eur. J. Med. Chem. 206, 112526. https://doi.org/10.1016/j.ejmech.2020.112526 (2020).
Article CAS PubMed Google Scholar
Negi, M., Chawla, P. A., Faruk, A. & Chawla, V. Role of heterocyclic compounds in SARS and SARS CoV-2 pandemic. Bioorg. Chem. 104, 104315. https://doi.org/10.1016/j.bioorg.2020.104315 (2020).
Article CAS PubMed PubMed Central Google Scholar
Girgis, A. S. et al. 3-Alkenyl-2-oxindoles: Synthesis, antiproliferative and antiviral properties against SARS-CoV-2. Bioorg. Chem. 114, 105131. https://doi.org/10.1016/j.bioorg.2021.105131 (2021).
Article CAS PubMed PubMed Central Google Scholar
Di Sarno, V. et al. Identification of a dual acting SARS-CoV-2 proteases inhibitor through in silico design and step-by-step biological characterization. Eur. J. Med. Chem. 226, 113863. https://doi.org/10.1016/j.ejmech.2021.113863 (2021).
Article CAS PubMed PubMed Central Google Scholar
Zhang, G.-N. et al. Discovery and optimization of 2-((1H-indol-3-yl)thio)-N-benzylacetamides as novel SARS-CoV-2 RdRp inhibitors. Eur. J. Med. Chem. 223, 113622. https://doi.org/10.1016/j.ejmech.2021.113622 (2021).
Article CAS PubMed PubMed Central Google Scholar
Zhao, J. et al.2-((1H-indol-3-yl)thio)-N-phenyl-acetamides: SARS-CoV-2 RNA-dependent RNA polymerase inhibitors.Antiviral Res.196 , 105209. https://doi.org/10.1016/j.antiviral.2021.105209 (2021).
Article CAS PubMed PubMed Central Google Scholar
Hattori, S.-I. et al. A small molecule compound with an indole moiety inhibits the main protease of SARS-CoV-2 and blocks virus replication. Nat. Commun. 12, 668. https://doi.org/10.1038/s41467-021-20900-6 (2021).
Article CAS PubMed PubMed Central Google Scholar
Shin, Y. S. et al. Discovery of cyclic sulfonamide derivatives as potent inhibitors of SARS-CoV-2. Bioorg. Med. Chem. Lett. 31, 127667. https://doi.org/10.1016/j.bmcl.2020.127667 (2021).
Article CAS PubMed Google Scholar
Dhama, K. et al. SARS-CoV-2 jumping the species barrier: Zoonotic lessons from SARS, MERS and recent advances to combat this pandemic virus. Trav. Med. Infect. Dis. 37, 101830. https://doi.org/10.1016/j.tmaid.2020.101830 (2020).
Srour, A. M. et al. Synthesis of aspirin-curcumin mimic conjugates of potential antitumor and anti-SARS-CoV-2 properties. Bioorg. Chem. 117, 105466. https://doi.org/10.1016/j.bioorg.2021.105466 (2021).
Article CAS PubMed PubMed Central Google Scholar
Power, H. et al. Virtual screening and in vitro validation of natural compound inhibitors against SARS-CoV-2 spike protein. Bioorg. Chem. 119, 105574. https://doi.org/10.1016/j.bioorg.2021.105574 (2022).
Article CAS PubMed Google Scholar
Chen, H., Cheng, F. & Li, J. iDrug: Integration of drug repositioning and drug-target prediction via cross-network embedding. PLoS Comput. Biol. 16, e1008040. https://doi.org/10.1371/journal.pcbi.1008040 (2020).
Article ADS CAS PubMed PubMed Central Google Scholar
https://www.drugs.com/history/paxlovid.html.
https://go.drugbank.com/unearth/q?utf8=%E2%9C%93&searcher=drugs&query=Paxlovid.
https://go.drugbank.com/drugs/DB15661.
https://www.drugs.com/history/molnupiravir.html.
Gougis, P. et al. Anticancer drugs and COVID-19 antiviral treatments in patients with cancer: What can we safely use?. Eur. J. Cancer 136, 1–3. https://doi.org/10.1016/j.ejca.2020.05.027 (2020).
Article CAS PubMed PubMed Central Google Scholar
Aldea, M., Michot, J.-M., Danlos, F.-X., Ribas, A. & Soria, J.-C. Repurposing of anticancer drugs expands possibilities for antiviral and anti-inflammatory discovery in COVID-19. Cancer Discov. 11, 1336–1344. https://doi.org/10.1158/2159-8290.CD-21-0144 (2021).
Article CAS PubMed Google Scholar
Díaz-Carballo, D. et al. Therapeutic potential of antiviral drugs targeting chemorefractory colorectal adenocarcinoma cells overexpressing endogenous retroviral elements. J. Exp. Clin. Cancer Res. 34, 81. https://doi.org/10.1186/s13046-015-0199-5 (2015).
Article CAS PubMed PubMed Central Google Scholar
Zhu, Z. et al. Arbidol monotherapy is superior to lopinavir/ritonavir in treating COVID-19. J. Infect. 81, e21–e23. https://doi.org/10.1016/j.jinf.2020.03.060 (2020).
Article CAS PubMed PubMed Central Google Scholar
Deng, L. et al. Arbidol combined with LPV/r versus LPV/r alone against corona virus disease 2019: A retrospective cohort study. J. Infect. 81, e1–e5. https://doi.org/10.1016/j.jinf.2020.03.002 (2020).
Article CAS PubMed PubMed Central Google Scholar
Li, Y. et al. Efficacy and safety of Lopinavir/Ritonavir or Arbidol in adult patients with mild/moderate COVID-19: An exploratory randomized controlled trial. Med 1, 105–113. https://doi.org/10.1016/j.medj.2020.04.001 (2020).
Xu, P. et al. Arbidol/IFN-α2b therapy for patients with corona virus disease 2019: A retrospective multicenter cohort study. Microbes Infect. 22, 200–205. https://doi.org/10.1016/j.micinf.2020.05.012 (2020).
Article CAS PubMed PubMed Central Google Scholar
Wei, S., Xu, S. & Pan, Y.-H. Efficacy of arbidol in COVID-19 patients: A retrospective study. World J. Clin. Cases 9, 7350–7357. https://doi.org/10.12998/wjcc.v9.i25.7350 (2021).
Article PubMed PubMed Central Google Scholar
Fawzy, N. G. et al. Novel curcumin inspired antineoplastic 1-sulfonyl-4-piperidones: Design, synthesis and molecular modeling studies. Anti-Cancer Agents Med. Chem. 19, 1069–1078. https://doi.org/10.2174/1871520619666190408131639 (2019).
Fawzy, N. G. et al. Synthesis, human topoisomerase IIα inhibitory properties and molecular modeling studies of anti-proliferative curcumin mimics. RSC Adv. 9, 33761–33774. https://doi.org/10.1039/c9ra05661k (2019).
Article ADS CAS PubMed PubMed Central Google Scholar
https://www.cancer.gov/about-cancer/treatment/drugs/fluorouracil.
https://www.cancer.gov/about-cancer/treatment/drugs/fluorouracil-topical.
https://www.drugs.com/history/sutent.html.
https://www.cancer.gov/about-cancer/treatment/drugs/sunitinibmalate.
Wödlinger, M. et al. Automated identification of cell populations in flow cytometry data with transformers. Comput. Biol. Med. 144, 105314. https://doi.org/10.1016/j.compbiomed.2022.105314 (2022).
Wopereis, S. et al. Evaluation of ER, PR and HER2 markers by flow cytometry for breast cancer diagnosis and prognosis. Clin. Chim. Acta 523, 504–512. https://doi.org/10.1016/j.cca.2021.11.005 (2021).
Article CAS PubMed Google Scholar
Nam, G.-H. et al. An optimized protocol to determine the engulfment of cancer cells by phagocytes using flow cytometry and fluorescence microscopy. J. Immunol. Methods 470, 27–32. https://doi.org/10.1016/j.jim.2019.04.007 (2019).
Article CAS PubMed Google Scholar
Riccardi, C. & Nicoletti, I. Analysis of apoptosis by propidium iodide staining and flow cytometry. Nat. Protoc. 1, 1458–1461. https://doi.org/10.1038/nprot.2006.238 (2006).
Article CAS PubMed Google Scholar
Propidium Iodide Flow Cytometrykit for cycle analysis, ab139418 (http://www.abcam.com).
Annexin V-FITC Apoptosis Detection Kit (Catalog#K101-25), BioVision, CA 94043 USA (http://www.biovision.com).
Cheng, M. et al. Discovery of potent and selective epidermal growth factor receptor (EGFR) bifunctional small-molecule degraders. J. Med. Chem. 63, 1216–1232. https://doi.org/10.1021/acs.jmedchem.9b01566 (2020).
Article CAS PubMed PubMed Central Google Scholar
Kilic, O. et al. Anti-EGFR fibronectin bispecific chemically self-assembling nanorings (CSANs) induce potent T cell-mediated antitumor responses and downregulation of EGFR signaling and PD-1/PD-L1 expression. J. Med. Chem. 63, 10235–10245. https://doi.org/10.1021/acs.jmedchem.0c00489 (2020).
Article CAS PubMed Google Scholar
Mishani, E. et al. High-affinity epidermal growth factor receptor (EGFR) irreversible inhibitors with diminished chemical reactivities as positron emission tomography (PET)-imaging agent candidates of EGFR overexpressing tumors. J. Med. Chem. 48, 5337–5348. https://doi.org/10.1021/jm0580196 (2005).
Article CAS PubMed Google Scholar
Zhang, Y. et al. Discovery of novel potent VEGFR-2 inhibitors exerting significant antiproliferative activity against cancer cell lines. J. Med. Chem. 61, 140–157. https://doi.org/10.1021/acs.jmedchem.7b01091 (2018).
Article CAS PubMed Google Scholar
Hasegawa, M. et al. Discovery of novel benzimidazoles as potent inhibitors of TIE-2 and VEGFR-2 tyrosine kinase receptors. J. Med. Chem. 50, 4453–4470. https://doi.org/10.1021/jm0611051 (2007).
Article CAS PubMed Google Scholar
Bhide, R. S. et al. Discovery and preclinical studies of (R)-1-(4-(4-fluoro-2-methyl-1H-indol-5-yloxy)-5-methylpyrrolo[2,1-f][1,2,4]triazin-6-yloxy)propan-2-ol (BMS-540215), an in vivo active potent VEGFR-2 inhibitor. J. Med. Chem. 49, 2143–2146. https://doi.org/10.1021/jm051106d (2006).
Article CAS PubMed Google Scholar
Wissner, A. et al. 2-(Quinazolin-4-ylamino)-[1,4]benzoquinones as covalent-binding, irreversible inhibitors of the kinase domain of vascular endothelial growth factor receptor-2. J. Med. Chem. 48, 7560–7581. https://doi.org/10.1021/jm050559f (2005).
Article CAS PubMed Google Scholar
Santa Cruz Biotechnology, Inc., EGFR (528): sc-120 (http://www.scbt.com).
Santa Cruz Biotechnology, Inc., VEGFR2 (A-3): sc-6251 (http://www.scbt.com).
Zang, J. et al. Discovery of novel pazopanib-based HDAC and VEGFR dual inhibitors targeting cancer epigenetics and angiogenesis simultaneously. J. Med. Chem. 61, 5304–5322. https://doi.org/10.1021/acs.jmedchem.8b00384 (2018).
Article CAS PubMed Google Scholar
Fu, R.-G., Sun, Y., Sheng, W.-B. & Liao, D.-F. Designing multi-targeted agents: An emerging anticancer drug discovery paradigm. Eur. J. Med. Chem. 136, 195–211. https://doi.org/10.1016/j.ejmech.2017.05.016 (2017).
Article CAS PubMed Google Scholar
Seliem, I. A. et al. New pyrazine conjugates: Synthesis, computational studies, and antiviral properties against SARS-CoV-2. ChemMedChem 16, 3418–3427. https://doi.org/10.1002/cmdc.202100476 (2021).
Article CAS PubMed PubMed Central Google Scholar
Çakır, V. & Arslan, T. Synthesis and biological evaluation of new silicon(IV) phthalocyanines as carbonic anhydrase and cholinesterase inhibitors. Inorg. Chim. Acta 530, 120678. https://doi.org/10.1016/j.ica.2021.120678 (2022).
Ullah, R. et al. Attenuation of spatial memory in 5xFAD mice by targeting cholinesterases, oxidative stress and inflammatory signaling using 2-(hydroxyl-(2-nitrophenyl)methyl)cyclopentanone. Int. Immunopharm. 100, 108083. https://doi.org/10.1016/j.intimp.2021.108083 (2021).
Benazzouz-Touami, A. et al. New coumarin-pyrazole hybrids: Synthesis, docking studies and biological evaluation as potential cholinesterase inhibitors. J. Mol. Struct. 1249, 131591. https://doi.org/10.1016/j.molstruc.2021.131591 (2022).
Li, X. et al. Development of multifunctional primidinylthiourea derivatives as potential anti-Alzheimer agents. J. Med. Chem. 59, 8326–8344. https://doi.org/10.1021/acs.jmedchem.6b00636 (2016).
Article CAS PubMed Google Scholar
Malek, R. et al. New dual small molecules for Alzheimer’s disease therapy combining histamine H3 receptor (H3R) antagonism and calcium channels blockade with additional cholinesterase inhibition. J. Med. Chem. 62, 11416–11422. https://doi.org/10.1021/acs.jmedchem.9b00937 (2019).
Article CAS PubMed Google Scholar
Elsinghorst, P. W., Tanarro, C. M. G. & Gütschow, M. Novel heterobivalent tacrine derivatives as cholinesterase inhibitors with notable selectivity toward butyrylcholinesterase. J. Med. Chem. 49, 7540–7544. https://doi.org/10.1021/jm060742o (2006).
Article CAS PubMed Google Scholar
Zhan, G. et al. Amaryllidaceae alkaloids with new framework types from Zephyranthes candida as potent acetylcholinesterase inhibitors. Eur. J. Med. Chem. 127, 771–780. https://doi.org/10.1016/j.ejmech.2016.10.057 (2017).
Article CAS PubMed Google Scholar
Andrade, M. T. et al. Indole alkaloids from Tabernaemontana australis (Müell. Arg) Miers that inhibit acetylcholinesterase enzyme. Bioorg. Med. Chem. 13, 4092–4095. https://doi.org/10.1016/j.bmc.2005.03.045 (2005).
Article CAS PubMed Google Scholar
Chigurupati, S. et al. Identification of novel acetylcholinesterase inhibitors: Indolopyrazoline derivatives and molecular docking studies. Bioorg. Chem. 67, 9–17. https://doi.org/10.1016/j.bioorg.2016.05.002 (2016).
Article CAS PubMed Google Scholar
Atanasova, M. et al. Galantamine derivatives with indole moiety: Docking, design, synthesis and acetylcholinesterase inhibitory activity. Bioorg. Med. Chem. 23, 5382–5389. https://doi.org/10.1016/j.bmc.2015.07.058 (2015).
Article CAS PubMed Google Scholar
Kia, Y., Osman, H., Kumar, R. S., Basiri, A. & Murugaiyah, V. Ionic liquid mediated synthesis of mono- and bisspirooxindole-hexahydropyrrolidines as cholinesterase inhibitors and their molecular docking studies. Bioorg. Med. Chem. 22, 1318–1328. https://doi.org/10.1016/j.bmc.2014.01.002 (2014).
Article CAS PubMed Google Scholar
Girgis , AS , Tala , SR , Oliferenko , PV , Oliferenko , AA & Katritzky , AR Computer-assisted rational design, synthesis, and bioassay of non-steroidal anti-inflammatory agents .Eur.J. Med.Chem.50 , 1–8https://doi.org/10.1016/j.ejmech.2011.11.034 (2012).
Article CAS PubMed Google Scholar
Girgis, A. S. et al. Synthesis, and QSAR analysis of anti-oncological active spiro-alkaloids. Org. Biomol. Chem. 13, 1741–1753. https://doi.org/10.1039/c4ob02149e (2015).
Article CAS PubMed Google Scholar
http://www.codessa-pro.com/manuals/manual.htm.
This work was supported financially by National Research Centre, Egypt, project ID: 12060101.
Open access funding provided by The Science, Technology & Innovation Funding Authority (STDF) in cooperation with The Egyptian Knowledge Bank (EKB).
Department of Pesticide Chemistry, National Research Centre, Dokki, Giza, 12622, Egypt
Nehmedo G. Fawazy, Mohamed S. Bekheit, Reham F. Barghash & Adel S. Girgis
Department of Chemistry and Physics, Augusta University, Augusta, GA, 30912, USA
Center of Scientific Excellence for Influenza Viruses, National Research Centre, Giza, 12622, Egypt
Ahmed Mostafa, Yassmin Moatasim & Omnia Kutkat
School of Chemistry, Cardiff University, Main Building, Park Place, Cardiff, CF10 3AT, UK
Drug Bioassay-Cell Culture Laboratory, Pharmacognosy Department, National Research Centre, Dokki, Giza, 12622, Egypt
Walid Fayad, May A. El-Manawaty & Ahmed AF Soliman
Department of Pharmacognosy, Faculty of Pharmacy, Cairo University, Cairo, 11562, Egypt
Department of Therapeutic Chemistry, National Research Centre, Dokki, Giza, 12622, Egypt
You can also search for this author in PubMed Google Scholar
You can also search for this author in PubMed Google Scholar
You can also search for this author in PubMed Google Scholar
You can also search for this author in PubMed Google Scholar
You can also search for this author in PubMed Google Scholar
You can also search for this author in PubMed Google Scholar
You can also search for this author in PubMed Google Scholar
You can also search for this author in PubMed Google Scholar
You can also search for this author in PubMed Google Scholar
You can also search for this author in PubMed Google Scholar
You can also search for this author in PubMed Google Scholar
You can also search for this author in PubMed Google Scholar
You can also search for this author in PubMed Google Scholar
You can also search for this author in PubMed Google Scholar
N.G.F., M.S.B., A.M.S. and R.F.B.: performed the synthetic studies; A.M., Y.M. and O.K.: performed the antiviral studies. B.M.K.: performed the X-ray studies; W.F., M.A.E. and A.A.F.S.: performed the antitumor studies. R.A.E.: performed the cholinesterase studies; A.S.G. and S.S.P.: designed the study, analyzed, interpreted the data and wrote the paper. All authors reviewed the manuscript.
Correspondence to Adel S. Girgis.
The authors declare no competing interests.
Springer Nature remains neutral with regard to jurisdictional claims in published maps and institutional affiliations.
Open Access This article is licensed under a Creative Commons Attribution 4.0 International License, which permits use, sharing, adaptation, distribution and reproduction in any medium or format, as long as you give appropriate credit to the original author(s) and the source, provide a link to the Creative Commons licence, and indicate if changes were made. The images or other third party material in this article are included in the article's Creative Commons licence, unless indicated otherwise in a credit line to the material. If material is not included in the article's Creative Commons licence and your intended use is not permitted by statutory regulation or exceeds the permitted use, you will need to obtain permission directly from the copyright holder. To view a copy of this licence, visit http://creativecommons.org/licenses/by/4.0/.
Fawazy, N.G., Panda, S.S., Mostafa, A. et al. Development of spiro-3-indolin-2-one containing compounds of antiproliferative and anti-SARS-CoV-2 properties. Sci Rep 12, 13880 (2022). https://doi.org/10.1038/s41598-022-17883-9
DOI: https://doi.org/10.1038/s41598-022-17883-9
Anyone you share the following link with will be able to read this content:
Sorry, a shareable link is not currently available for this article.
Provided by the Springer Nature SharedIt content-sharing initiative
By submitting a comment you agree to abide by our Terms and Community Guidelines. If you find something abusive or that does not comply with our terms or guidelines please flag it as inappropriate.
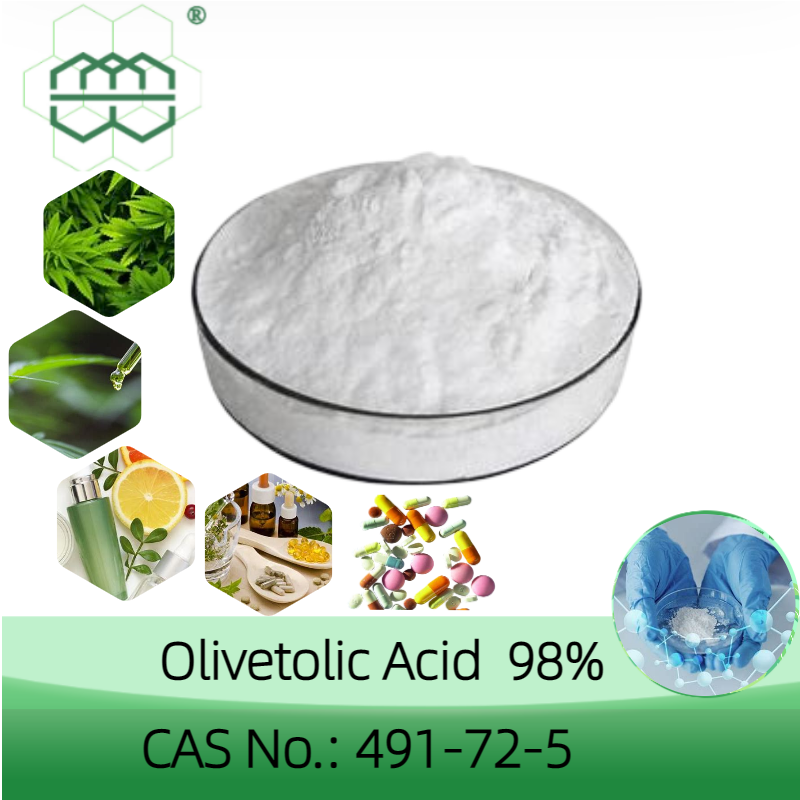
Disodium Adenosine Triphosphate Scientific Reports (Sci Rep) ISSN 2045-2322 (online)